Exploring Bosons: Key Particles in Quantum Physics
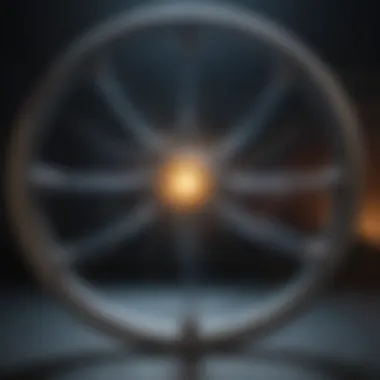
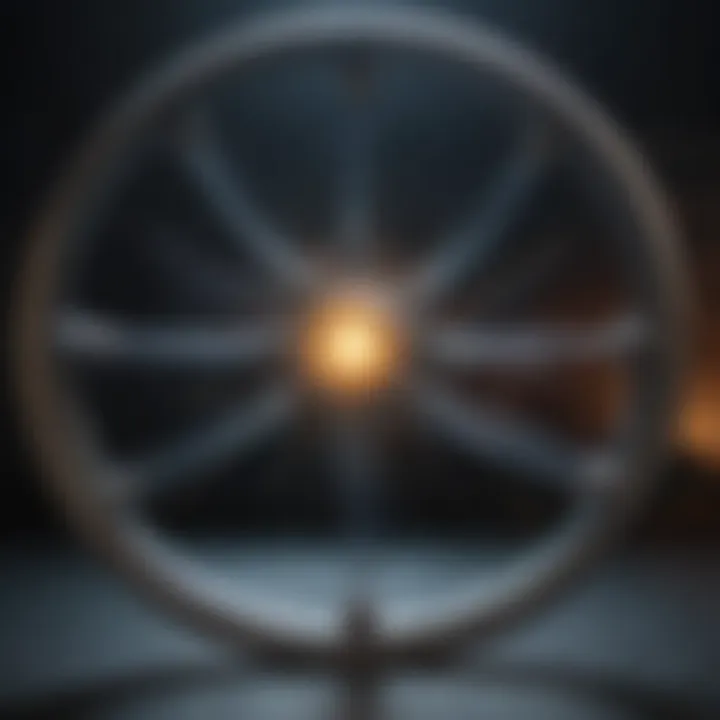
Intro
Bosons play a crucial role in the world of quantum physics. These particles, distinct from their fermion counterparts, possess unique characteristics that lend them significant importance within the framework of the standard model of particle physics. Understanding bosons requires diving into their fundamental properties, classifications, and the forces they mediate.
At their core, bosons are integer-spin particles, meaning they follow a different set of statistical rules compared to fermions, which obey the Pauli exclusion principle. Their nature allows them to occupy the same quantum state, facilitating various phenomena in the universe. This article will take a closer look at the various types of bosons, their roles in the fundamental forces of nature, and the experimental methods researchers use to detect them.
As we venture deeper, we'll unpack why studying bosons matters not just for physicists, but also for our wider understanding of the universe. The journey through this intricate topic promises to illuminate the complexities surrounding these fundamental particles and their implications for contemporary physics.
Defining Bosons and Their Role in Physics
Understanding bosons is like finding the missing piece in a vast cosmic puzzle. These fundamental particles not only play a crucial role in the universe's structure but also help us grasp the very fabric of reality. At the heart of many physical interactions, bosons are responsible for mediating fundamental forces and influences in the subatomic world. When one thinks about how particles behave, it's essential to comprehend the distinct characteristics that bosons hold.
What Are Bosons?
Bosons can be thought of as the workhorses of the quantum world. Unlike their more rambunctious counterparts, fermions, bosons follow different rules. They are particles that have an integer spin, which affects how they maintain their collective existence. Essentially, this attribute enables multiple bosons to occupy the same quantum state without hindrance. For instance, consider how photons can exist in a single beam of light—this wouldn’t be feasible if they were fermions.
Bosons are classified based on their roles, often seen as "force carriers" in the universe. Take photons, for example, they carry electromagnetic force. Likewise, the W and Z bosons are responsible for weak nuclear force, while gluons hold the strong force responsible for binding quarks together. The Higgs boson, on the other hand, relates to mass generation through the Higgs mechanism, illuminating why some particles have mass while others remain massless.
Bosons vs. Fermions: A Comparative Analysis
A clear distinction exists between bosons and fermions, each exhibiting unique properties and behaviors:
- Spin Characteristics:
- Collective Behavior:
- Examples:
- Bosons possess integer spins (0, 1, 2), allowing them to bunch together in the same state.
- Fermions have half-integer spins (1/2, 3/2) and follow the Pauli exclusion principle, preventing them from occupying the same quantum state.
- Bosons can create phenomena such as Bose-Einstein condensation, where particles clump together at low temperatures, acting as a single quantum entity.
- Fermions maintain individuality, resulting in behaviors that align with statistical mechanics.
- Common bosons include photons, gluons, W/Z bosons, and the Higgs boson.
- Notable fermions include electrons, protons, and neutrons, forming the matter we see around us.
In summary, bosons serve pivotal roles that complement the actions of fermions. Their inherent traits make them indispensable in understanding not just atomic and subatomic interactions, but also the overarching principles governing the universe.
"Bosons are the gentle architects of the quantum realm, turning abstract forces into tangible interactions."
By defining bosons and contrasting them with fermions, we dismantle intricacies that lay the groundwork for deeper explorations in particle physics. The marvel of how these particles interact leads us into further investigations, igniting curiosity about our universe.
The Standard Model of Particle Physics
The Standard Model of particle physics stands as a monumental framework that exquisitely describes the fundamental constituents of matter and their interactions. It serves as a cornerstone in the landscape of modern physics, offering profound insights into how elementary particles— which include both fermions and bosons—come together to form the building blocks of the universe. This model is not merely a theoretical construct; it’s backed by extensive experimental verification, making it crucial for understanding the intricate workings of the cosmos.
One of the appealing features of the Standard Model is its efficiency. It succinctly categorizes all known elementary particles and their interactions through a clear set of principles. This classification simplifies the complexity of the universe, allowing scientists to make precise predictions about the outcomes of particle collisions observed in high-energy experiments. Additionally, the model elucidates critical concepts, such as symmetry and gauge invariance, essential for understanding fundamental physics.
Another significant aspect of the Standard Model lies in its incorporation of three of the four known fundamental forces: electromagnetism, the weak nuclear force, and the strong nuclear force. Gravity remains outside its purview, a factor that gives rise to ongoing debates and research in theoretical physics aimed at unifying all fundamental forces.
In essence, the Standard Model doesn’t just sit on a pedestal; it informs our understanding of the universe, shaping the discourse in both academic and applied physics. Its impact can be seen in various fields, including particle astrophysics and cosmology.
Foundation of the Standard Model
The foundation of the Standard Model is rooted in particle field theory, which describes how particles behave through quantum fields. At its core lies the concept of quantum chromodynamics (QCD) and electroweak theory, serving as the key pillars that hold this model together.
The Components
The model’s framework is built upon two distinct classes of particles:
- Fermions: These particles make up matter. They include quarks (which form protons and neutrons) and leptons (such as electrons).
- Bosons: These are force carriers that mediate interactions between fermions, including the photon for electromagnetic force, W and Z bosons for the weak force, and gluons for the strong force.
Together, these particles interweave to create the complex tapestry of visible matter and the forces that operate within and between them.
Theoretical Underpinnings
The mathematical descriptions used in the Standard Model revolve around group symmetries, providing a concerted method to represent interactions. Gauge theories are prominent here, where the forces correspond to the symmetries of the underlying fields. This mathematical rigor allows for predictions, such as the behaviors of particles during high-energy collisions.
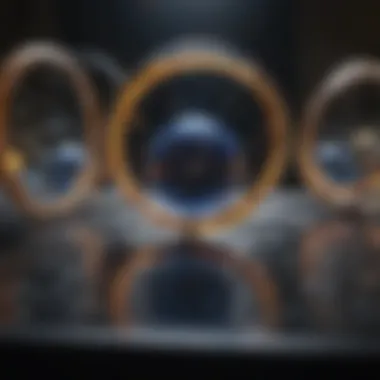
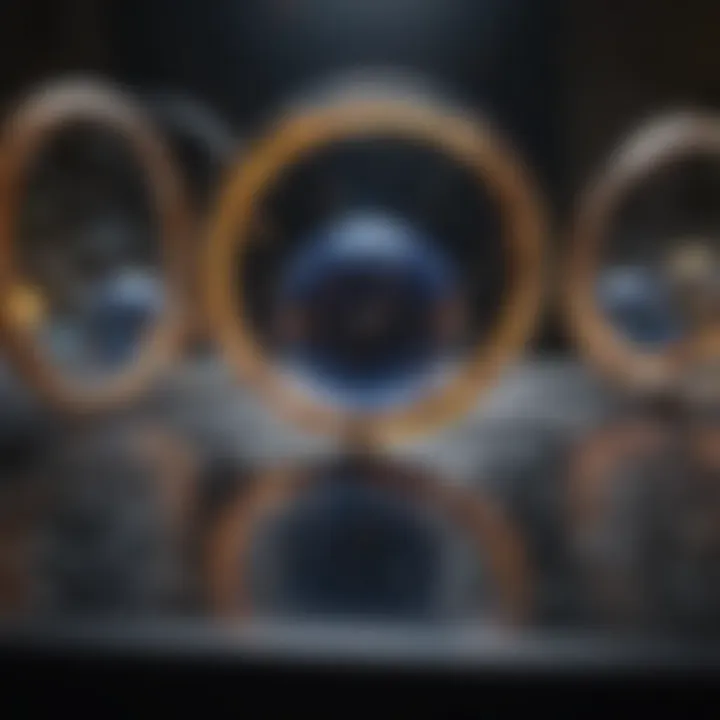
Moreover, one can’t ignore the significance of the Higgs mechanism in this paradigm. It introduces mass into the particle physics arena, explaining how elementary particles acquire their mass through interaction with the Higgs field—something that was further validated with the experimental discovery of the Higgs boson at the Large Hadron Collider.
Integration of Bosons in the Standard Model
Bosons play a pivotal role within the Standard Model. Their integration not only facilitates the understanding of forces at a microscopic level but also reinforces the underlying principles that govern particle interactions.
Mechanisms of Interaction
Bosons are crucial in transmitting forces between particles. For instance, the photon is tasked with mediating electromagnetic interactions. Whenever charged particles interact, they do so by exchanging photons, an act fundamental to electricity and magnetism.
Similarly, the W and Z bosons facilitate the weak nuclear force, which is responsible for processes such as beta decay. Gluons, on the other hand, bind quarks together within protons and neutrons, effectively holding the atomic nucleus intact. Each of these bosons unlocks a different facet of interactions, shaping our understanding of how particles behave across various scales.
"The Standard Model elegantly captures the interplay between matter and forces, yet remains incomplete."
Future Considerations
The understanding of bosons and their integration into the Standard Model not only deepens theoretical insights but also motivates experimental inquiries. Physicists continually search for phenomena that elude the current model, such as dark matter and the gravitational force. This pursuit cultivates a fertile ground for new theories, pushing the boundaries of what the Standard Model can explain.
In wrapping up the discussion on the importance of the Standard Model, it becomes evident that it’s an essential building block for comprehensive physics. Its synthesis of bosons illustrates the elegance of fundamental interactions, while simultaneously opening avenues for exciting research and discovery.
Types of Bosons
Understanding the classification of bosons is vital in quantum physics, as these particles have roles that range from mediating fundamental forces to shaping our understanding of the universe at the smallest scales. By exploring the various types of bosons, we not only appreciate their unique characteristics but also gain insights into how they interact within the framework of the Standard Model. Here’s a detailed exploration of two main categories of bosons: gauge bosons and scalar bosons.
Gauge Bosons: Force Carriers
Gauge bosons are the key players when it comes to force mediation in particle physics. They are responsible for the fundamental interactions in nature—gravitational, electromagnetic, weak, and strong forces. Let’s delve into the specifics of these force carriers.
Photon
The photon is perhaps the most well-known of all bosons, serving as the force carrier for electromagnetic interactions. Its importance can't be overstated; this particle is responsible for transmitting light and other forms of electromagnetic radiation across space.
A standout characteristic of the photon is its unique property of being massless. This allows photons to travel at the speed of light, making them extremely efficient carriers of energy and information. In practical terms, this means that every time we turn on a light, we're witnessing the behavior of countless photons flashing to and fro.
However, there are downsides as well. The nature of photons means they only interact with electrically charged particles. Thus, while they play a critical role in countless phenomena—such as photosynthesis or the technology behind solar panels—they're not involved in strong or weak force interactions, which limits their scope.
W and Z Bosons
The W and Z bosons are pivotal when it comes to mediating the weak nuclear force, and they are essential for processes like beta decay. These particles possess mass, unlike the photon, which results in a very short range for the weak interaction. They operate behind the scenes in processes involving nuclear reactions.
What makes these bosons particularly fascinating is their dual role. The W bosons come in two varieties, W+ and W-, and they can change the type of particle they interact with, which is crucial in particle transformations. The Z boson, on the other hand, carries no charge and is responsible for neutral current interactions. This feature of W and Z bosons, having mass while also facilitating such transformative interactions, makes them vital for understanding how particles interact under weak nuclear forces.
However, their heavy mass leads to short-lived interactions, which can pose challenges when studying them in experimental settings. Thus, while they are fundamental to processes that occur in the universe, their study requires robust facilities like particle colliders.
Gluon
Gluons are another vital category of gauge boson, specifically responsible for mediating the strong nuclear force. This is the force that binds quarks together, and consequently the particles that make up protons and neutrons. A particularly remarkable trait of gluons is that they carry a type of charge known as "color charge," which is a fundamental property in quantum chromodynamics (QCD).
One of the unique features of gluons is their ability to bind with each other due to this color charge. This self-interaction leads to complex dynamics that make gluons essential for understanding the stability of atomic nuclei. In simpler terms, gluons are the glue that holds matter together at its most fundamental level.
However, studying gluons is not without difficulties. The complexity involved in their interactions demands advanced theories and high energies found in particle accelerators. Their role in forming the structure of matter emphasizes their necessity in particle physics research.
Scalar Bosons: The Higgs Boson
While gauge bosons are crucial for mediating forces, scalar bosons play a different but equally significant role. Among them, the Higgs boson stands out as a critical element of the Standard Model. The Higgs field that associates with this boson is vital for understanding why other particles have mass. The discovery of the Higgs boson in 2012 was a significant milestone in confirming this aspect of modern physics and has massive implications on our understanding of particle interactions.
In essence, the study of bosons, both gauge and scalar, is key to grasping the fundamental workings of the universe. As researchers continue to investigate these types, they pave the way for potential discoveries that could reshape our understanding of physics.
The Discovery of the Higgs Boson
The discovery of the Higgs boson stands as one of the pivotal moments in the field of particle physics. It not only reinforces the framework of the Standard Model but also opens the door to profound questions about the essence of mass and the universe's structure. Without the Higgs boson, we would face a significant gap in our understanding of how particles acquire mass, a fundamental concept that impacts theories ranging from the mundane to the cosmic.
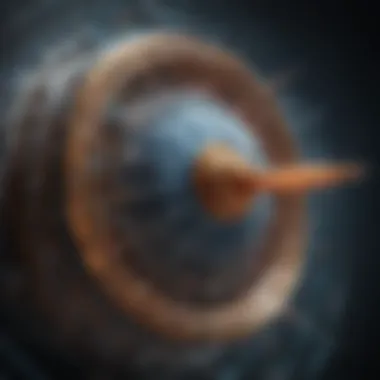
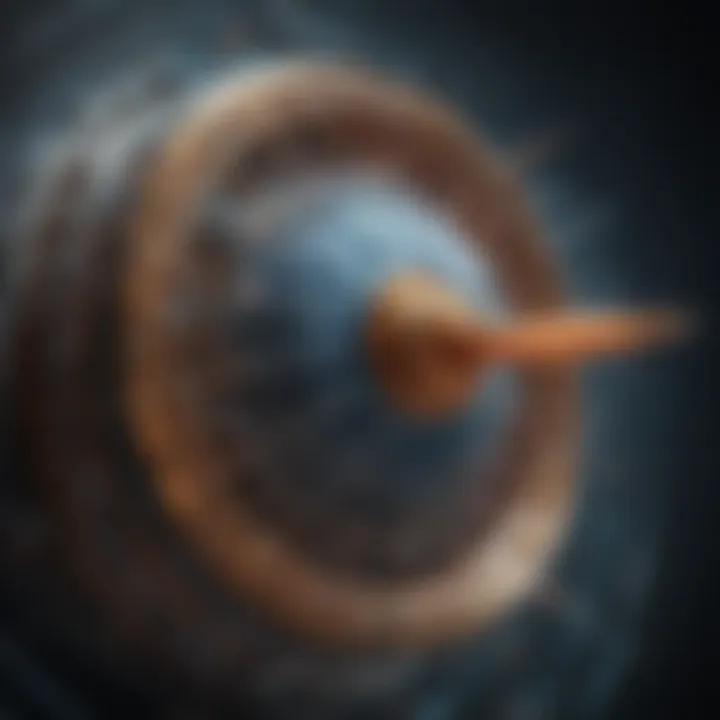
Background of the Higgs Mechanism
The Higgs mechanism is central to understanding particle mass in the quantum world. This fascinating process comes from the field proposed by physicist Peter Higgs in the 1960s, which states that a field permeates the universe, allowing particles to gain mass through their interactions with it. When particles move through this Higgs field, they experience a sort of drag, much like trying to walk through water. The extent of this interaction determines a particle's mass.
This theoretical framework predicted the existence of the Higgs boson, a particle associated with the field. Its discovery at CERN confirmed the theory and allowed physicists to validate the Standard Model with empirical evidence.
Experiments Leading to Discovery
CERN and the Large Hadron Collider
CERN, the European Organization for Nuclear Research, has been a leading institute in particle physics since its establishment. It is renowned for the Large Hadron Collider (LHC), the world's most powerful particle accelerator, located underground near Geneva. The LHC is unique because it can accelerate protons to nearly the speed of light and smash them together. These high-energy collisions recreate conditions similar to those just after the Big Bang.
The LHC's key characteristic is its vast size and capabilities, allowing for numerous experiments under extreme conditions. Researchers can study tiny particles and their interactions in unprecedented detail. One might say it's a giant microscope for subatomic particles.
Moreover, the LHC has a distinctive feature: it is equipped with sophisticated detectors like ATLAS and CMS, which meticulously capture data from particle collisions. This aspect is fundamentally advantageous as it enables scientists to sift through vast amounts of data to find rare occurrences, such as the creation of the Higgs boson.
Implications of Discovery
The implications of discovering the Higgs boson extend far beyond just confirming the Standard Model. Recognizing the Higgs particle has transformed our understanding of several areas in physics. For instance, it refocuses discussions on dark matter and energy as well as possible extensions to the Standard Model, such as supersymmetry.
One key characteristic of the discovery’s implications is that it bridges theoretical physics with experimental confirmation, shaping future research. Researchers are now better equipped to formulate hypotheses about the universe's underlying mechanics, pushing the boundaries beyond what the Standard Model can explain.
In summary, the Higgs boson's discovery holds immense significance. It solidifies the foundational principles of particle physics while prompting new explorations in realms previously deemed mysterious. With every new experiment and theory, our comprehension of the universe grows deeper, shedding light on phenomena we have yet to fully understand.
Experimental Techniques in Boson Research
The exploration of bosons is not merely an academic exercise; it directly contributes to our understanding of the universe at a fundamental level. Within this realm, the experimental techniques employed are crucial, as they enable researchers to unveil the properties and interactions of these fundamental particles. The effective study of bosons hinges upon various sophisticated methods that harness enormous energy levels and advanced detection capabilities. Up next, we delve deeper into the core components of this work—colliders and particle detection, followed by the intricacies involved in data analysis and interpretation.
Colliders and Particle Detection
Colliders stand as monumental feats of engineering and scientific ingenuity. These vast structures accelerate particles to near-light speeds, facilitating head-on collisions that recreate conditions similar to those just after the Big Bang. The collisions yield enormous energy, allowing for the fleeting existence of bosons, which typically decay almost instantly. The Large Hadron Collider (LHC) at CERN is a prime example, utilizing a 27-kilometer tunnel to accelerate protons. It has been instrumental in the discovery of the Higgs boson and continues to contribute to our understanding of particle physics.
Key Aspects of Colliders:
- Energy Levels: Higher energy collisions can lead to the creation of heavier bosons.
- Collision Frequency: The number of collisions per second is essential for significant findings.
- Target Selection: Different target particles provide varied outcomes, yielding diverse data for analysis.
- Detector Systems: Unlike simple sensors, these systems must account for the highly charged environment created by fast-moving particles.
Particle detection is an art unto itself. Once bosons are produced during collisions, they often decay rapidly, transforming into other particles. The key lies in detecting the resultant particles and discerning their origins. Various detectors, such as the ATLAS and CMS, track and measure the energy and trajectories of these decay products. Through intricate designs featuring multiple layers of technology, these detectors capture critical data, offering insight into the properties of the original bosons.
Data Analysis and Interpretation
The real magic happens when the dust settles after a collision and researchers sift through the mountains of data generated. Every collision yields a plethora of potential outcomes, all needing meticulous analysis. Data analysis in boson research involves rigorous statistical methods to distinguish genuine particle signals from background noise—the remnants of myriad failed interactions.
The Steps of Data Analysis:
- Data Collection: This initial phase gathers extensive raw data from detector systems.
- Event Reconstruction: Sophisticated algorithms reconstruct particle trajectories and energy profiles.
- Signal Extraction: Identifying potential signals of bosons amid overwhelming background events.
- Statistical Modeling: Applying models to determine likelihoods for various particle interactions.
- Peer Validation: Ensuring findings undergo rigorous scrutiny from the scientific community to validate results.
This complex dance of data ultimately leads to interpretative models that explain the boson's role and properties in physics. By dissecting statistical outputs, scientists can unravel theories regarding the universe's structure and the fundamental forces at play.
“Understanding bosons can offer insights not just into the particles themselves but also into the fundamental laws that govern everything in our universe.”
The symbiosis between advanced colliders and astute data interpretation forms the backbone of contemporary boson research. As technology advances, the sensitivity and precision of these experimental techniques will continue to evolve, opening doors to new revelations and potentially groundbreaking discoveries in the field of particle physics.
Ongoing Research on Bosons
Ongoing research on bosons is pivotal to advancing our grasp of the universe. In this field, scientists endeavor to unravel the complexities related to fundamental particles. It not only contributes to the body of knowledge in particle physics but also has significant implications for technology and our understanding of the cosmos. The pursuit of knowledge in this area can lead to innovative approaches in various domains, from telecommunications to medical applications. Thus, grasping the subtleties of ongoing boson research becomes essential.
Exploring Beyond the Standard Model
Supersymmetry and Bosons
Supersymmetry presents a compelling theoretical framework where bosons and fermions are interconnected. This theory posits that every particle has a superpartner, which opens the door to new insights in cosmology and particle behavior. The key characteristic of supersymmetry is its potential to address several puzzles in particle physics, such as the hierarchy problem.
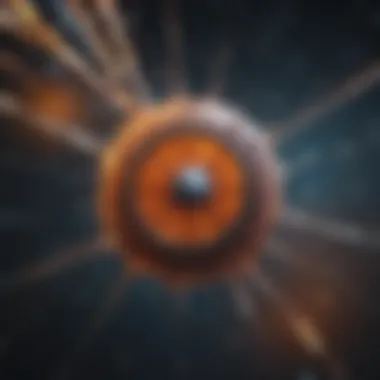
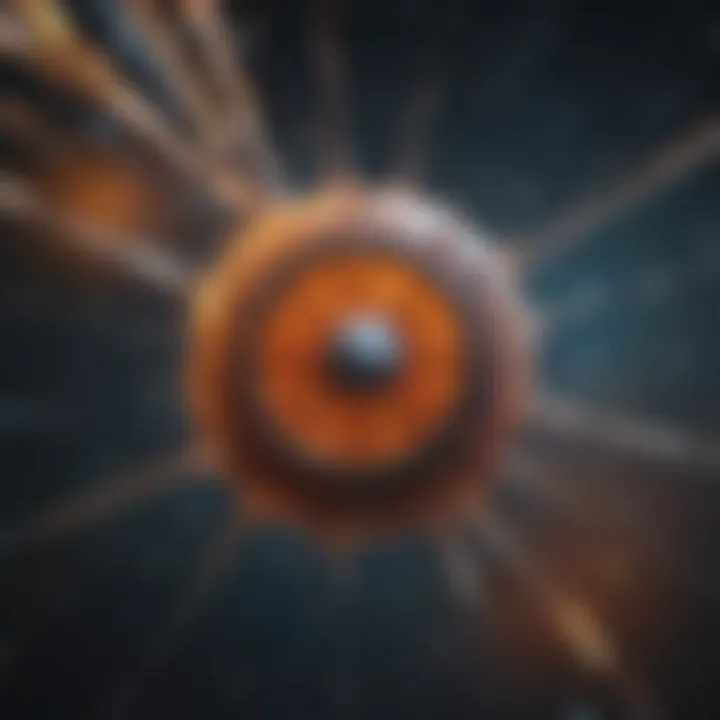
Why is this concept favored in the realm of boson research? Fundamentally, it offers a coherent extension to the Standard Model, providing viable candidates for dark matter and enhancing the overall structure of particle physics. The unique feature here is the prediction of new bosonic states, such as the hypothetical scalar boson, which could offer answers to questions currently troubling physicists.
However, there are challenges. Experimental evidence for supersymmetry has been elusive, leading some to question its validity. Yet, the pursuit continues, as the benefits could revolutionize our understanding of the universe.
Possible New Bosonic Particles
New bosonic particles beyond the known ones, like W, Z, and gluons, pose intriguing possibilities. Concepts such as axions and dilatons are part of the speculative landscape in particle physics. Their existence could lead to explanations of dark energy and dark matter, which remain some of the biggest mysteries of our time.
The primary characteristic of these alleged new particles is their potential to unify forces and explain phenomena unexplained by the current understanding. They could also lead to advances in quantum field theory and cosmology. The allure of these particles is their role in piecing together the cosmos's puzzle.
Unique to these discussions is the fact that while they could offer great advantages in terms of theoretical richness and experimental exploration, they also pose significant risks. Theoretical uncertainty is high, and the experiments to detect such particles can be exceedingly challenging.
Current Experiments and Future Directions
Current experiments play a crucial role in validating theories about bosons and exploring the nature of those particles. Institutions like CERN lead the charge, with the Large Hadron Collider at the forefront of particle collisions aiming to uncover new physics. These experiments analyze interactions at high energies, hoping to provide empirical evidence supporting or refuting the hypotheses surrounding bosons.
">"Cutting-edge research in this field not only pushes the boundaries of known physics but also paves the way for future technological innovations and deeper understandings."
As we look to the future, the road ahead is paved with possibility. Advances in experimental techniques, such as improved detectors and innovative collision strategies, are set to broaden our horizons further. The next decade promises exciting opportunities to discover and understand new bosonic particles, including those theorized ones that could drastically reshape our theoretical landscape.
In summary, ongoing research on bosons reflects more than just scientific inquiry; it represents humanity's innate desire to comprehend and exploit the fabric of the cosmos. As scientists pursue these lines of inquiry, they inch closer to deciphering the mysteries of existence.
Practical Applications of Boson Research
The realm of boson research stretches far beyond theoretical physics; it provides tangible benefits and innovative applications that permeate various sectors of society. By studying these fundamental particles, we not only deepen our understanding of the universe but also uncover advancements that enhance technology and improve quality of life. This section explores how boson research influences practical applications, emphasizing two key areas: technology and medical imaging.
Impacts on Technology and Industry
Bosons play a critical role in several technological innovations that drive industries today. These particles, particularly in the context of gauge bosons like photons, serve as the backbone of various technologies. For instance, the development of quantum computing heavily relies on principles that govern bosons. Here are a few aspects of their impact:
- Communication: Photons, as gauge bosons, are essential for fiber optic communications. They allow data to travel at the speed of light, revolutionizing how we transmit information over long distances.
- Data Storage: The principles of bosons contribute to the advancement of quantum bits, or qubits, which are foundational in the storage and processing of information in quantum computers. This allows for processing capabilities far exceeding those of traditional binary systems.
- Material Science: Bosons help in understanding electromagnetic interactions, paving the way for new materials with unique properties. For instance, high-temperature superconductors have applications in energy transmission and storage, significantly reducing power loss.
The collaboration between physics and industry thus nurtures innovations that not only enhance efficiency but also reduce costs and environmental impacts across various sectors.
Enhancements in Medical Imaging
Another prominent area influenced by boson research is medical imaging. The exploration of bosons has led to developments that transform how we visualize and diagnose medical conditions. These advancements include:
- Positron Emission Tomography (PET): Using the principles of particle physics, particularly involving positrons—the antiparticles of electrons—PET scans allow for highly detailed images by detecting gamma rays produced during positron-electron annihilation. This technique significantly improves early cancer detection and monitoring.
- Magnetic Resonance Imaging (MRI): While not directly reliant on bosons, advances in the understanding of particle interactions under magnetic fields have enhanced MRI technologies. It provides clearer images of soft tissues compared to traditional methods, resulting in better diagnostic outcomes.
- Nanotechnology: Insights from boson research are paving the way for nanomedicine, where engineered nanoparticles are used for targeted drug delivery systems. This approach optimizes treatment efficiency and minimizes side effects.
In summary, the implications of boson research extend into our daily lives, from communications technology to groundbreaking developments in medicine. This fusion of fundamental physics with practical applications holds great promise for future advancements.
Through such explorations, we stand on the cusp of potentially transformative breakthroughs that not only deepen our understanding of the universe but also profoundly affect how we live and interact with technology.
Philosophical Implications of Boson Studies
The exploration of bosons extends beyond mere scientific inquiry; it dives into profound philosophical questions about the very fabric of our reality. Bosons, as fundamental particles, are not just carriers of forces but also keys to understanding the universe's underlying principles. In a sense, they challenge our perceptions of existence and interaction.
One significant aspect of studying bosons is how it reshapes our understanding of causality and the interconnectedness of matter and energy. Bosons, particularly gauge bosons, mediate the fundamental forces that shape everything from atomic structures to cosmic phenomena. When scientists investigate how these particles interact, they probe the nexus of cause and effect in a realm that often defies classical intuition.
The Nature of Reality in Quantum Physics
In quantum physics, the nature of reality appears far from what our daily experiences might lead us to believe. For instance, particles don't occupy defined states until measurement occurs, a concept that gives rise to numerous philosophical debates. Consider the role of the Higgs boson, essentially giving mass to other particles through its interactions. This interaction questions the foundational understanding of 'mass' itself: is it a property inherent to objects, or is it an emergent feature arising from particle interactions?
Moreover, the wave-particle duality that characterizes quantum entities directly impacts our philosophy. It suggests that, at a fundamental level, nature might possess qualities that are difficult, if not impossible, to reconcile with human intuition.
"Quantum physics challenges the distinction between observer and observed, collapsing - or not - the state of a particle. Thus, reality itself hinges on these interactions with bosons."
This leads us into deeper metaphysical waters. If reality hinges on interactions mediated by bosons, how do we conceptualize separation between objects? Are they truly distinct from one another, or are they manifestations of a more intricate interweaving? These considerations prompt researchers from various disciplines to ponder the implications of their work.
Challenges in Understanding the Quantum Realm
Navigating the quantum landscape is akin to wandering through a foggy maze. Even the most astute physicists face hurdles in grasping the implications of their findings. One key challenge lies in interpreting quantum effects at large scales. How can we apply principles that work well on a subatomic level to macroscopic phenomena?
Additionally, the interpretations of quantum mechanics—like the Copenhagen interpretation or many-worlds theory—present distinct philosophical quandaries. Take the observer effect, for instance: it raises questions about the role of consciousness in the measurement process. Does the mere act of observing a particle influence its behavior? These challenges not only bewilder but also compel scientists to rethink long-held assumptions.
To add to the complexity, the potential existence of new bosonic particles—ones that may not fit neatly into the Standard Model—further muddies the waters. As physicist David Gross noted, the "beauty of our theories often outpaces our understanding." This sentiment echoes throughout the boson discourse, reminding us that while we strive for clarity, the universe itself remains a richly layered tapestry woven with yet-unanswered questions.
In summary, delving into the philosophical implications of boson studies illuminates the broader significance of ongoing research and its profound impact on our understanding of existence. The limits of classical engagement fall away, and in their wake, we find a narrative that treasures inquiry, reflection, and perhaps the greatest mystery of all: the quantum essence of reality itself.