Exploring Quantum Computers: Key Components Revealed
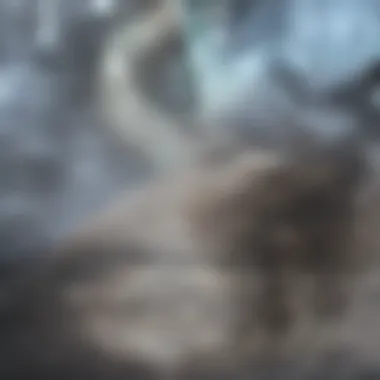
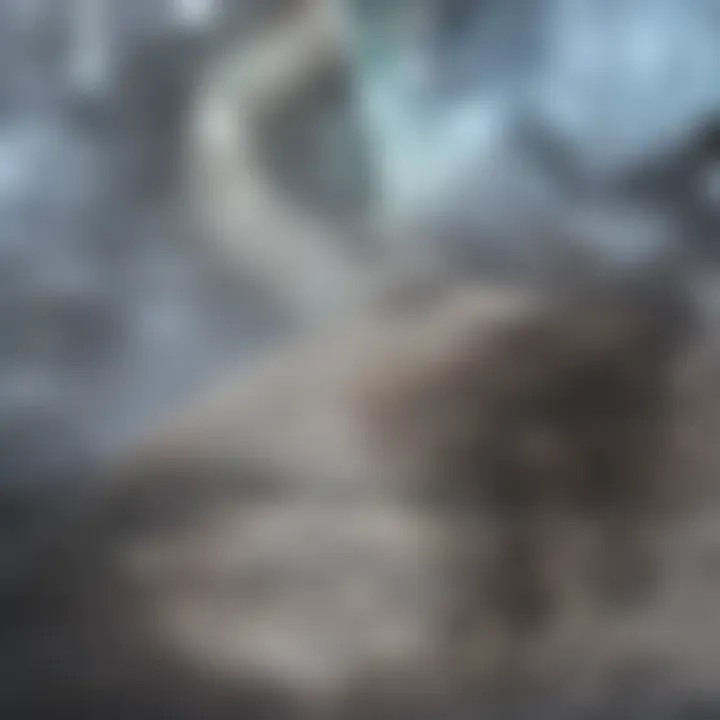
Intro
Quantum computing represents a significant leap forward in computing technology. Unlike classical computers, which use bits as the smallest unit of information, quantum computers utilize qubits. This distinct feature enables quantum computers to process a vast amount of data simultaneously and solve complex problems much quicker than their classical counterparts. Understanding the components that constitute quantum computers is essential for grasping how this revolutionary technology operates and its potential implications across various fields.
As we further explore the intricate components involved, we will focus on key elements such as qubits, quantum gates, and essential hardware. We will also consider how classical computing systems interface with quantum technology, which plays a crucial role in the development of effective quantum algorithms and applications. This overview serves as a comprehensive guide, delivering insights into the current advancements and challenges in the quantum computing landscape, tailored specifically for students, researchers, and professionals in the field.
"The power of quantum computers lies in their ability to perform complex calculations much faster than traditional computers."
To facilitate our discussion, this article will be structured methodically, covering foundational concepts followed by detailed examinations of the technical aspects that constitute quantum computing. The insights shared here aim to enrich the reader's understanding and appreciation of this cutting-edge technology.
Foreword to Quantum Computing
Quantum computing represents a profound shift in how we process information. With its roots embedded in quantum mechanics, this field promises to tackle problems that conventional computing struggles with. Understanding quantum computing is essential not just for technology enthusiasts, but also for professionals in numerous fields, including cryptography, pharmaceuticals, and artificial intelligence.
The components of quantum computers, including qubits and quantum gates, are vital to grasping their unique capabilities. Unlike classical bits, which are limited to two states (0 or 1), qubits can exist in multiple states simultaneously due to superposition. This property allows quantum computers to perform many calculations at once, potentially revolutionizing tasks such as cryptography and optimization problems.
Moreover, grasping the significance of quantum computing goes beyond its theoretical aspects. The advancements made in this field hold real-world implications for industries and society at large. As more organizations invest in quantum technologies, understanding this shift becomes crucial.
"Quantum computation involves using quantum-mechanical phenomena to perform operations on data." - OpenAI
In summary, the introduction to quantum computing not only outlines the theoretical foundations but also establishes the context for its application. As we navigate through the components of quantum computers, we will explore how these systems are built and the revolutionary changes they can bring.
Fundamental Elements of Quantum Computers
Quantum computers represent a paradigm shift in computation due to their unique elements. Understanding these fundamental components is crucial. It enables researchers and professionals to grasp how quantum computers function and the potential benefits they bring. Each component, from qubits to quantum gates, plays a distinct role that contributes to the overall efficiency and capability of quantum systems.
What is a Qubit?
A qubit, or quantum bit, is the basic unit of information in quantum computing. Unlike a classical bit, which can be either 0 or 1, a qubit can exist in a state of superposition. This means it can simultaneously hold both values until measured. This characteristic allows quantum computers to process a vast amount of information concurrently. The manipulation of qubits is at the heart of quantum computations, making their understanding essential.
Types of Qubits
Qubits can be implemented in various ways, each offering unique advantages and challenges. The main types of qubits include superconducting qubits, ion trap qubits, and topological qubits. Each type contributes differently to the performance and stability of quantum systems.
Superconducting Qubits
Superconducting qubits are known for their scalability and speed. They use superconducting circuits that operate at temperatures near absolute zero. This characteristic allows them to achieve a high degree of coherence, which is critical for maintaining quantum states.
Their key feature is the ability to form complex circuits on chip, making them a popular choice among researchers.
Advantages:
- High operational speed.
- Improved scalability due to chip integration.
Disadvantages:
- Requires cryogenic temperatures.
- Vulnerability to noise challenges.
Ion Trap Qubits
Ion trap qubits use electrically charged atoms, or ions, confined in electromagnetic fields. This method benefits from precise control and long coherence times. Because they can be manipulated using lasers, it allows for excellent accuracy in quantum operations.
The unique feature is their inherent capability to maintain quantum information over time effectively.
Advantages:
- High fidelity and long coherence times.
- Robustness against certain types of errors.
Disadvantages:
- Complexity in scaling up systems.
- Requires precise laser control.
Topological Qubits
Topological qubits are considered an emergent design that relies on anyonic states in condensed matter systems. Their stability arises from topological properties, making them resistant to local disturbances. This characteristic can potentially reduce errors in quantum operations significantly.
Advantages:
- Intrinsically fault-tolerant.
- Potential for easier scalability.
Disadvantages:
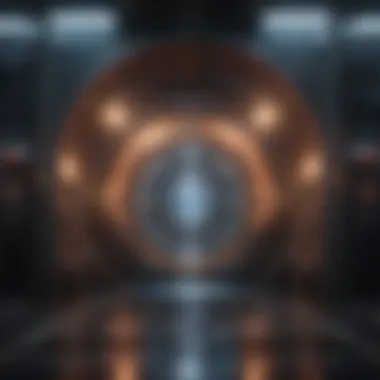
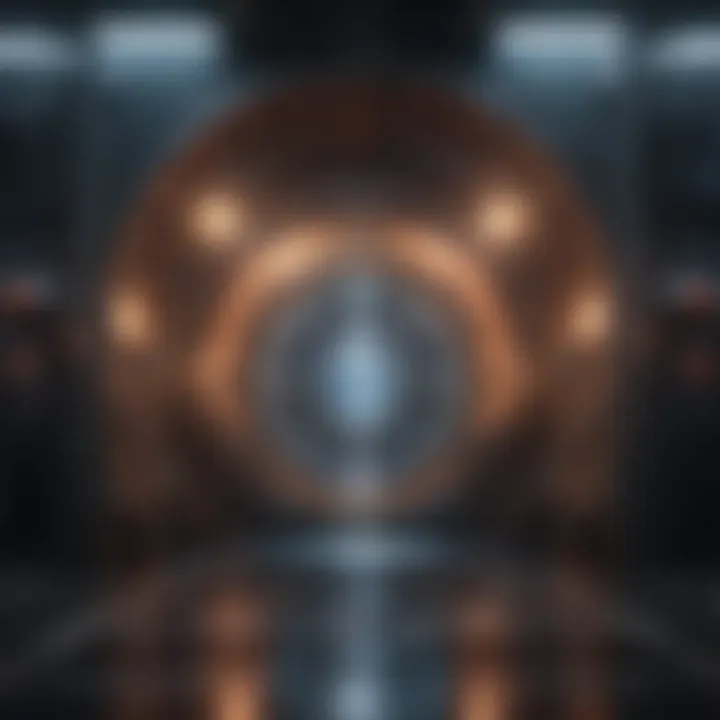
- Still in early research stages.
- Limited practical implementations.
Quantum Gates Explained
Quantum gates are the building blocks of quantum circuits. They manipulate the states of qubits and allow for the execution of quantum algorithms. Understanding the functions and types of quantum gates is critical for anyone studying quantum computing.
Single-Qubit Gates
Single-qubit gates affect one qubit at a time. They rotate the qubit's state around the Bloch sphere, allowing for complex computations. These gates are fundamental to any quantum algorithm since they provide the means to change a qubit from one state to another.
Their ease of implementation makes them beneficial for many quantum applications.
Advantages:
- Simplicity in design and execution.
- Essential for more complex quantum algorithms.
Disadvantages:
- Limited to manipulating only one qubit at a time.
Two-Qubit Gates
Two-qubit gates enable interactions between pairs of qubits, facilitating entanglement—a crucial aspect of quantum computing. The most commonly used two-qubit gates include the CNOT gate. These gates are vital as they allow quantum systems to leverage entanglement, thus enhancing computational power.
Advantages:
- Helps create entangled states.
- Enables more complex quantum logic operations.
Disadvantages:
- Often more complex to implement than single-qubit gates.
Measurement Gates
Measurement gates are used to observe the state of qubits. This process collapses the superposition of a qubit into either a 0 or 1. The ability to measure qubits accurately is essential since it determines the outcome of quantum computations. Measurement gates introduce classical information into quantum systems, bridging the quantum and classical environments.
Advantages:
- Essential for extracting information from quantum computations.
- Allows verification of quantum states.
Disadvantages:
- Could introduce errors due to the collapse of superpositions.
- Measurement can disrupt the system's quantum state.
Understanding these fundamental components of quantum computers provides a framework for further exploration into their capabilities and applications. The distinctions among qubits and quantum gates illuminate how quantum technologies are poised to revolutionize computing.
Supportive Hardware in Quantum Computing
Supportive hardware plays a critical role in the functionality of quantum computers. Unlike classical computers, quantum systems operate under principles of quantum mechanics that necessitate specific conditions for their efficient and reliable operation. Understanding supportive hardware is essential because it underlines the constraints and capabilities of quantum computation, directly influencing the performance of quantum algorithms.
Control Electronics
Control electronics are essential for managing the operations of qubits. They provide the necessary control signals that manipulate the state of qubits during computations. These electronics must operate with extreme precision. Any slight error can lead to significant computational inaccuracies. The development of these electronics requires advanced circuits and signal processing techniques. They are also paramount in converting digital signals into analog signals that qubits can use.
High-speed operation is crucial in this area, and engineers often employ field-programmable gate arrays (FPGAs) to create custom hardware solutions for these tasks. The control electronics must work seamlessly with qubits to realize the full potential of quantum computation. Therefore, a deep understanding of these systems is vital for anyone engaged in quantum research and development.
Cryogenic Systems
For quantum computers to function correctly, they must operate at extremely low temperatures. Cryogenic systems are necessary to maintain these conditions. Cooling the quantum processor minimizes thermal noise, which can interfere with the delicate states of qubits. Hardware such as dilution refrigerators is common in quantum labs. These systems cool components to near absolute zero, often around 10mK or colder.
The importance of cryogenic systems cannot be overstated. They not only create a suitable environment for qubits but also impact the overall system's energy consumption and stability. Designing efficient cooling solutions continues to be a challenge, as the expense and complexity of maintaining these low temperatures can be significant. Understanding cryogenic technology is thus crucial for researchers and engineers working in quantum computing.
Quantum Error Correction Mechanisms
Quantum error correction is a fundamental aspect of making quantum computations reliable. Unlike classical bits, qubits are susceptible to errors from decoherence and noise. To tackle these issues, a variety of quantum error correction codes have been developed. These mechanisms work by encoding the information of a single logical qubit into multiple physical qubits. This redundancy allows the system to detect and correct errors without measuring the qubits directly, which would collapse their quantum states.
Key error correction codes include the Shor code and the Steane code, each designed to protect against different types of errors. Implementing effective quantum error correction is vital for building scalable quantum computers. Overall, understanding these mechanisms is crucial for advancing the field of quantum technology.
"Quantum error correction is to quantum computing what error correction is to classical computing; without it, the road to practical quantum computers would be fraught with challenges."
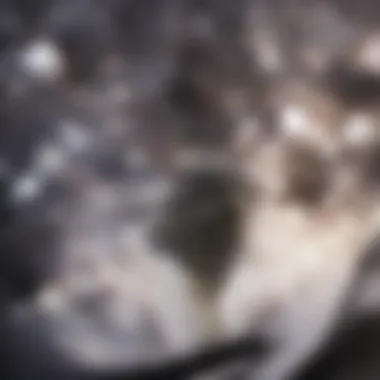
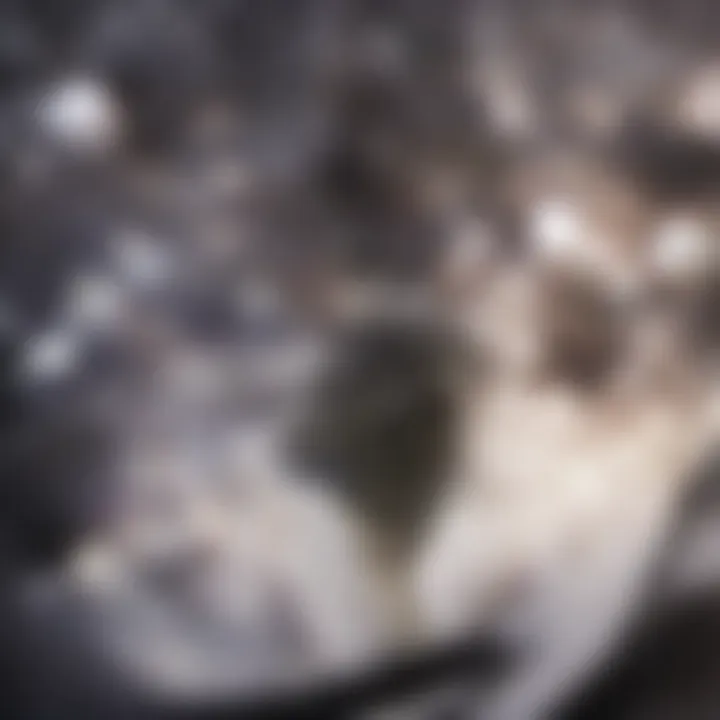
Quantum Algorithms and Protocols
Quantum algorithms and protocols serve as the backbone of quantum computing, guiding how operations are performed within these systems. As quantum computers process information in fundamentally different ways compared to classical computers, the development of specialized algorithms is vital. These algorithms take advantage of quantum mechanics, enabling tasks that are impractical or impossible for classical systems. Understanding these algorithms is crucial for grasping the full potential of quantum technology, including its advantages in various fields such as cryptography, optimization, and data analysis.
Overview of Quantum Algorithms
Quantum algorithms are designed to exploit the principles of superposition and entanglement. Unlike classical algorithms, which rely on bits as the smallest unit of information, quantum algorithms manipulate qubits. A qubit can represent a 0, a 1, or both at the same time, allowing for parallel computations. This unique feature leads to increased efficiency in certain computations.
The importance of quantum algorithms lies in their potential to solve problems that classical computers struggle with. For example, some algorithms can factor large numbers exponentially faster than classical methods, which has significant implications for security. Moreover, the creation of quantum protocols improves communication security, illustrating the real-world applications of this technology.
Key Algorithms in Quantum Computing
Shor's Algorithm
Shor's Algorithm is fundamental in demonstrating the power of quantum computing. Its main purpose is to solve integer factorization, a task that poses significant challenges for classical computers, particularly regarding large prime numbers. The key characteristic of Shor's Algorithm is its polynomial time complexity, enabling it to factor large numbers swiftly, which can compromise current encryption methods.
What makes Shor's Algorithm a pivotal subject in this article is its ability to highlight the potential risks to classical cryptography. A notable feature of Shor's Algorithm is that it uses quantum Fourier transform, which is efficient for detecting periodicity in numbers. This advantage enables it to break widely used cryptographic systems like RSA.
However, there are challenges associated with Shor's Algorithm. Quantum computers capable of executing it efficiently are not yet fully realized, posing questions about practical implementation. Nonetheless, its theoretical implications resonate throughout quantum technology discussions.
Grover's Algorithm
Grover's Algorithm provides another significant contribution to the realm of quantum computing. Its goal is to search through an unsorted database more quickly than any classical algorithm can. The key aspect of Grover's Algorithm is that it offers a quadratic speedup, reducing the time needed to search from O(N) to O(√N).
This makes Grover's Algorithm beneficial for many scenarios, including data analysis and security, where rapid search capabilities are paramount. The unique feature of Grover's Algorithm lies in its use of amplitude amplification, which increases the probability of finding the correct solution during each iteration.
However, there are also disadvantages. The speedup provided by Grover's Algorithm, while significant, is not exponential, making it less revolutionary than Shor's Algorithm in some contexts. Yet, it remains essential for understanding how quantum computing can improve search algorithms in classical systems.
"Quantum algorithms represent a paradigm shift in computational approaches, offering new solutions to age-old problems."
The exploration of these algorithms sheds light on the transformative potential of quantum computing, emphasizing the necessity of continued research and development in this exciting field.
Integrating Classical and Quantum Systems
Quantum computing offers a paradigm shift in how we approach problem-solving. However, to fully leverage the capabilities of quantum mechanics, it is essential to integrate classical computing systems effectively. Classical computing remains central in providing a framework for quantum technologies. This section explores the roles both systems play and how their collaboration enhances computational power and performance.
Role of Classical Computing
Classical computers are still vital in quantum computing for various reasons. They perform tasks that involve large datasets and processes that do not require quantum efficiency. Some key roles include:
- Control Systems: Classical computers manage the operations of quantum bits, or qubits. They orchestrate the initialization, manipulation, and measurement of qubits during computations.
- Data Processing: Data generated from quantum calculations often needs further classical processing. This includes interpreting results and feeding back into quantum algorithms for refinement.
- Error Correction: Classical systems contribute significantly to error correction methods in quantum computing. By monitoring qubits, they can help rectify errors caused by decoherence and noise, enhancing the reliability of quantum systems.
The interaction between classical and quantum systems is fundamental. Classical systems process information that quantum computers generate. Their synergy is necessary for improving the user experience and ensuring broader applications of quantum technologies.
Hybrid Quantum Classical Systems
The notion of hybrid systems arises from the need to merge classical and quantum computing efficiently. These systems capitalize on the strengths of both paradigms to overcome the limitations inherent in each.
- Architecture Design: Hybrid systems often have an architecture that allows seamless interaction between classical and quantum components. This design is crucial for developing efficient algorithms that can run on both types of hardware.
- Enhanced Performance: By leveraging classical components, hybrid systems can run complex quantum algorithms that classical systems find challenging. The classical part can handle large data management tasks, while the quantum section executes the parallel processing capabilities.
- Software Development: The development of software platforms that can operate across both classical and quantum systems is essential. Tools such as Qiskit and Cirq enable developers to create applications that exploit the unique capabilities of quantum computers while still relying on classical resources for certain computations.
Integrating classical and quantum systems is not just about running quantum hardware. It's about fostering collaboration between two vastly different computing paradigms, paving the way for significant advancements in the computing landscape. Ultimately, this integration positions us closer to fully realizing the practical benefits of quantum technology in real-world applications.
Emerging Technologies in Quantum Computing
Emerging technologies in quantum computing represent a critical frontier in the evolution of this field. As researchers explore new ways to enhance the capabilities of quantum systems, these technologies bring both exciting opportunities and unique challenges. Innovations in qubit design and error correction mechanisms are particularly noteworthy. They are not only reshaping our understanding of quantum information processing but also influencing practical applications that can emerge from these advancements.
Advancements in Qubit Design
Improvements in qubit design are paramount for the practical realization of quantum computers. Different types of qubits, such as superconducting qubits, ion trap qubits, and topological qubits, are under continuous investigation. Each type presents distinct advantages and trade-offs.
Superconducting qubits, for instance, leverage the properties of materials that exhibit zero electrical resistance at cryogenic temperatures. This feature allows them to be manipulated with high precision, which is essential for quantum computations. In recent years, advancements have focused on reducing the error rates associated with these qubits. Techniques like pulse optimization and improved circuit designs are yielding promising results.
Ion trap qubits, on the other hand, utilize ions trapped in electromagnetic fields. Their long coherence times make them an attractive option for quantum algorithms. Research into miniaturization and integration of these systems is ongoing, aimed at creating more scalable solutions.
Topological qubits are another intriguing avenue of research. They promise increased resistance to errors due to their unique properties. However, further development is necessary before they can be implemented widely in quantum systems. Thus, advancements in qubit design are critical not only for improving current quantum computers but also for paving the way for more robust quantum technologies in future.
Innovations in Quantum Error Correction
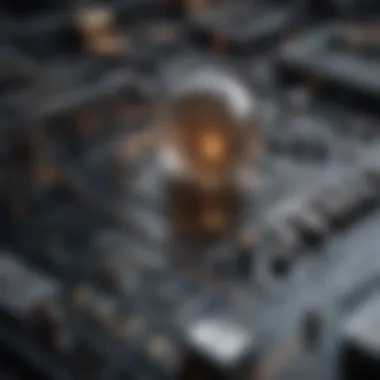
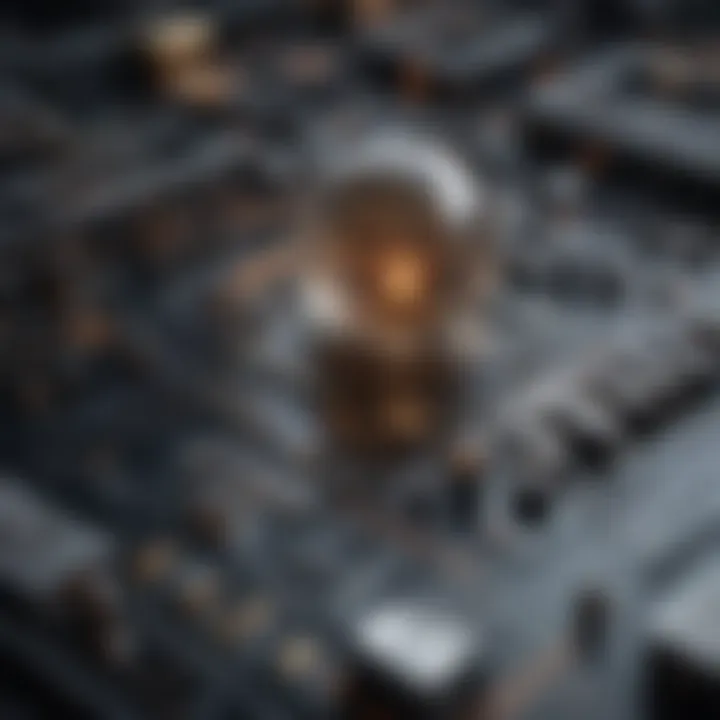
An equally important aspect of advancements in quantum computing is the innovation in error correction methods. Quantum systems are inherently prone to errors from decoherence and noise. Therefore, robust error correction techniques are essential to their functionality and performance.
Recent approaches in quantum error correction focus on encoding information in a way that protects it against errors. Logical qubits, which are constructed by entangling multiple physical qubits, provide a means to achieve this. Techniques such as surface codes and cat codes are examples of promising strategies that scientists are developing.
Furthermore, research is progressing on real-time error correction mechanisms. These methods aim to detect and correct errors as they occur, improving the overall reliability of quantum computations. The integration of classical processing power with quantum systems today is also key. It allows for more complex error correction protocols, ultimately leading to more efficient computation.
Challenges in Quantum Computing
Quantum computing stands at the forefront of technological advancement, yet it is not without its hurdles. These challenges pose significant implications for the scalability, efficiency, and practicality of quantum computers. Understanding these challenges is essential for researchers, developers, and anyone interested in the future of computing. This section will elaborate on two main issues: decoherence and noise, followed by scalability concerns. Together, they form a core part of the ongoing dialogue surrounding quantum technologies.
Decoherence and Noise Issues
Decoherence refers to the loss of quantum coherence, wherein qubits lose their quantum state through interactions with their environment. This process is detrimental because it disrupts the delicate superpositions that qubits rely on for performing complex calculations. The primary sources of decoherence include thermal noise, electromagnetic interference, and interactions with stray particles.
Managing decoherence is crucial. Strategies such as quantum error correction have been developed to combat this issue. However, these techniques also require additional qubits, complicating the overall system. Noise complicates the scenario even further. While noise can originate from many external factors, it can also arise internally through imperfections in the qubit systems themselves. This variability in performance leads to error rates that must be accounted for when designing quantum algorithms.
"To harness the full potential of quantum computing, we must develop robust methods to mitigate the effects of decoherence and noise.”
Scalability Concerns
Scalability represents another formidable challenge in quantum computing. As quantum computers evolve, the need for increasing numbers of qubits becomes apparent. Each additional qubit introduces new errors, necessitating more sophisticated error correction mechanisms.
The current quantum architectures cannot yet support large-scale systems efficiently. While small test systems might operate effectively, extrapolating these results to a larger scale reveals significant performance decay due to error rates and decoherence. As such, the quest for scalability involves not only the design of more qubits but also the integration of supportive technologies that facilitate error-free calculations.
Consider the following factors affecting scalability:
- Qubit Fidelity: Higher fidelity is essential for preserving quantum information over extended computations.
- Interconnectivity: Efficient communication between qubits is vital, as limited connectivity can hinder processing speed and effectiveness.
- Physical Limitations: Current physical systems used to create qubits have inherent constraints that limit how they can be scaled up.
In summary, challenges like decoherence, noise, and scalability are critical issues in the realm of quantum computing. Addressing these problems requires innovative approaches and continued research, making them a key focus for future advancements in the field.
Future Directions in Quantum Computing
The significance of future directions in quantum computing extends beyond mere technological advancements. An evolving landscape in this domain entails a deep dive into the potential achievements, current efforts, and theoretical explorations. Understanding these aspects is crucial for grasping how quantum computing might redefine computation circuits and align more closely with classical computing.
Quantum Supremacy
Quantum supremacy refers to a critical milestone in quantum computing, where a quantum computer performs a calculation that is infeasible for classical computers. This concept underlines the capabilities of quantum systems and ignites interest in further technological developments. The announcement by Google in 2019, claiming to achieve this milestone with its Sycamore processor, marks a significant turning point. Researchers are now focusing on validating this claim and exploring real-world applications of quantum supremacy. Tracking performance metrics is vital to push boundaries and understand how quantum mechanics can be harnessed in computing effectively.
Potential Applications
Emerging technologies in quantum computing open doors to various applications. Each field has its intricacies and promises transformative impacts.
Cryptography
Cryptography stands as a prime candidate for quantum application due to its reliance on complex calculations. Traditional encryption methods, such as RSA, hinge on the difficulty of factoring large numbers—a task where Shor's Algorithm can dramatically outperform classical methods. This unique feature offers a pathway for improved security measures. The potential for quantum computing to disrupt current cryptographic systems is both a concern and a motivating factor, urging advances in quantum-resistant algorithms and protocols.
Drug Discovery
In pharmaceuticals, quantum computing shows promise in simulating molecular interactions at unprecedented scales. This characteristic allows researchers to explore extensive chemical databases quickly. Coupled with machine learning, quantum algorithms can lead to more efficient drug design processes. Despite benefits, challenges remain. The high level of precision required in quantum states can complicate experimental outcomes. The road ahead involves striking a balance between the theoretical capabilities of quantum models and practical implementations in drug discovery.
Optimization Problems
Optimization problems exist in various industries, from finance to transportation. Quantum computing's potential to analyze vast combinations of variables simultaneously presents an effective solution for complex optimization challenges. D-Wave's quantum annealing technology offers insights into resolving such problems. However, leveraging quantum mechanics is not straightforward. The landscape is characterized by an evolving understanding of quantum behavior and its limitations. Thus, businesses must tread carefully while integrating these systems into existing frameworks.
Quantum computing has the potential to revolutionize entire fields, challenging our traditional paradigms through innovative solutions.
In sum, the future directions in quantum computing represent a confluence of possibility and challenge. The interplay among quantum supremacy, diverse applications, and overcoming technological barriers will ultimately define how effectively quantum computers are integrated into real-world scenarios.
End
The conclusion section serves as an important reflection on the content explored throughout this article. It encapsulates the significant insights into quantum computing. As we have discussed, quantum computers are an intricate amalgamation of various components that work harmoniously to push computational boundaries. Understanding these elements is essential not only for theoretical knowledge but also for practical applications in various fields.
Summary of Key Points
In this article, we have covered several crucial aspects of quantum computing:
- Definition and Historical Context: The evolution of quantum computers from basic theories to practical applications showcases their potential.
- Fundamental Elements: Quibits and quantum gates are the backbone of quantum information processing. Their beenfits and varieties were explored in depth.
- Supportive Hardware: Control electronics and cryogenic systems create a robust environment for qubits, preventing decoherence and improving reliability.
- Quantum Algorithms: Algorithms such as Shor's and Grover's demonstrate the power of quantum computation, addressing complex problems efficiently.
- Integration with Classical Systems: The interplay between classical and quantum systems illustrates the ongoing transition within computing paradigms.
- Emerging Technologies and Challenges: Innovations in qubit design and error correction mechanisms are paving the way for a brighter future while addressing scalability and noise challenges.
- Future Directions: Topics like quantum supremacy and applications in cryptography, drug discovery, and optimization show the vast potential of quantum technology.
The Path Forward in Quantum Technology
Looking forward, the field of quantum technology presents numerous opportunities and challenges. Researchers are investigating various ways to enhance qubit performance, reduce error rates, and improve overall system stability. A considerable focus lies in developing scalable systems, which will allow quantum computers to tackle larger problems and operate more efficiently.
As quantum computing continues to mature, its applications in cryptography, complex problem-solving, and simulations in chemistry and physics will increasingly gain prominence. While the journey is not without obstacles, the prospects of quantum technology promise to radically reshape industries and scientific inquiry. Continued investment in research and collaboration among academia, industry, and governments will be crucial in realizing the full potential of quantum computing.
"The future of computing is not merely classical; it is quantum, and understanding its foundations will empower us to innovate."