Exploring Quantum Properties: An In-Depth Analysis
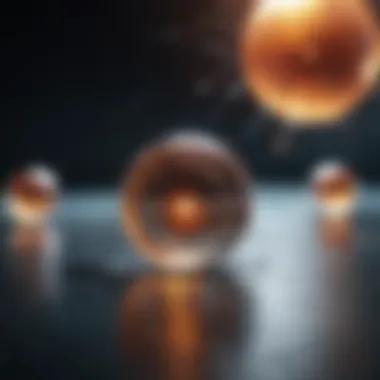
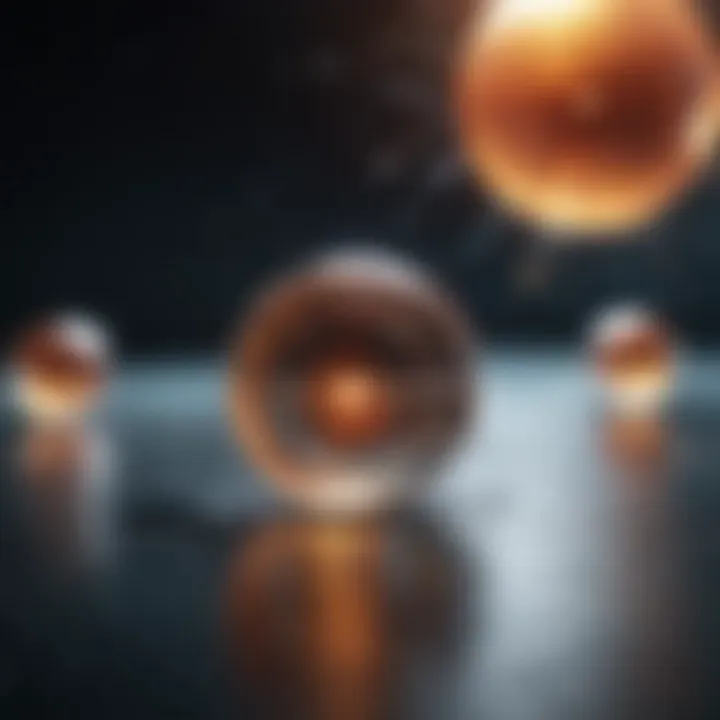
Intro
The study of quantum properties represents a vital branch of modern physics. Understanding the fundamental principles underlying matter and energy behavior at a microscopic level is essential for both theoretical and applied sciences. Concepts such as superposition, entanglement, and uncertainty are not merely theoretical musings; they have immense implications in various fields, including quantum computing, cryptography, and materials science. In this article, we will embark on a thorough exploration of these concepts.
By analyzing these quantum principles, we aim to establish the relevance they hold in today's technological advancements and scientific discourse. This exploration will not only inform but also inspire further inquiry into the vast potential these properties present.
Preface to Quantum Properties
Quantum properties are fundamental aspects of matter and energy that manifest at the microscopic level. Understanding these properties allows researchers and academics to explore the fabric of our universe. From the basic characteristics of particles to their interactions, each element of quantum phenomena holds significant implications for both theoretical and practical applications.
The exploration of quantum properties is imperative because it challenges traditional notions of classical physics. As we delve into these principles, it becomes clear how they not only shape our understanding of the microscopic world, but also impact technologies that govern everyday life. For instance, concepts such as superposition and entanglement have become the cornerstone for advancements in quantum computing and cryptography.
In this article, we will address several key areas related to quantum properties.
- We will clarify definitions and establish the scope of the topic.
- Historical context will be provided to highlight the evolution of these concepts.
- Key quantum phenomena such as wave-particle duality, superposition, and entanglement will be introduced.
- We will discuss differences between quantum mechanics and classical physics.
- Applications in various fields, including computing and cryptography, will be outlined.
- Existing challenges and theoretical implications will also be explored, such as decoherence and the measurement problem, alongside potential future research directions.
Our goal is to provide a cohesive understanding of quantum properties for students, researchers, educators, and professionals engaged in this crucial area of science. By emphasizing significant knowledge and considerations, we contribute to contemporary scientific discourse and inspire further inquiry into the realm of quantum mechanics.
Definition and Scope
Quantum properties can be defined as the distinctive features that characterize substances at atomic and subatomic levels. This includes phenomena that do not conform to classical intuitions about physics, such as the behavior of electrons around an atomic nucleus or the nature of light as both a wave and a particle. The scope of quantum properties encompasses several key concepts. By focusing on areas such as wave-particle duality, quantum superposition, and entanglement, this section lays a foundational understanding for the rest of the article. These are not merely theoretical curiosities—they have profound implications in technology, nature, and our comprehension of time and space.
Historical Context
The historical evolution of quantum mechanics is a tale of intellectual breakthroughs that redefined scientific thought. In the early 20th century, physicists like Max Planck and Albert Einstein initiated this journey. Planck introduced the concept of quantization of energy, leading to the birth of quantum theory, while Einstein's work on the photoelectric effect highlighted the particle-like behavior of light.
Subsequent developments in the 1920s by Niels Bohr and Werner Heisenberg further advanced the field. The Bohr model of the atom and Heisenberg's uncertainty principle were groundbreaking. Each theory represented a shift from deterministic physics to probabilistic interpretations of phenomena.
This section provides a brief overview of these milestones, illustrating how they contributed to our modern understanding of quantum mechanics. By exploring key historical milestones, readers will appreciate the continuous evolution of ideas that have led to contemporary research in quantum properties.
Fundamental Quantum Concepts
The study of quantum properties necessitates a solid grasp of fundamental quantum concepts. These concepts are not merely academic in nature; they serve as the foundation for understanding how matter and energy behave on a subatomic level. The significance lies in how these principles influence modern technologies and challenge classical understandings of physics. By delving into these fundamental ideas, researchers can unlock new possibilities for innovation and increase our comprehension of the universe's workings.
Wave-Particle Duality
Wave-particle duality is a cornerstone of quantum mechanics, highlighting the dual nature of light and matter. It suggests that entities such as electrons and photons exhibit both wave-like and particle-like characteristics depending on the experimental context. This concept begins with Thomas Young's double-slit experiment, where light creates an interference pattern, indicating its wave nature. Conversely, when photons are measured, they behave as particles.
Understanding wave-particle duality is crucial for fields like quantum optics, which explores how light interacts with matter. It also has practical implications in the development of photonic technologies, such as lasers and optical fibers. The duality nature challenges our classical intuitions, prompting a re-evaluation of fundamental physics concepts. Such re-evaluation can lead to novel applications in devices that utilize quantum properties.
Quantum Superposition
Quantum superposition describes the phenomenon where a quantum system can exist in multiple states at once, until it is measured. This principle is famously illustrated through Schrödinger's cat thought experiment, where a cat is both alive and dead until observed. Superposition allows quantum bits or qubits to represent both 0 and 1 simultaneously, providing a foundation for quantum computing.
The importance of quantum superposition extends beyond theoretical frameworks. It significantly increases computational power, enabling quantum computers to solve complex problems far quicker than classical computers. Industries that rely on massive data analysis will benefit dramatically from this quantum advantage. Explore more about superposition in quantum mechanics at Wikipedia.
Quantum Entanglement
Quantum entanglement occurs when two or more particles become linked, such that the state of one particle instantaneously influences the state of another, regardless of distance. This phenomenon defies classical concepts of locality and suggests that information can be shared faster than light speed, raising intriguing questions about the nature of space and time.
Entanglement forms the basis for several advanced technologies, including quantum cryptography, where it enhances security by ensuring that any attempt to intercept communication disturbs the quantum state, thus revealing eavesdropping. The implications of entanglement also extend to quantum teleportation, allowing for the transfer of quantum states across distances.
Quantum Uncertainty Principle
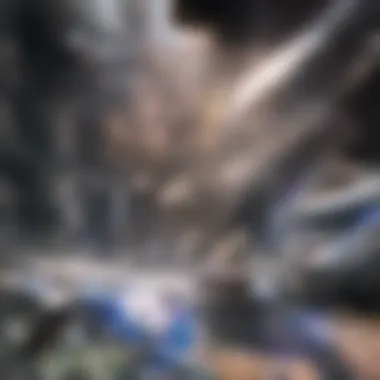
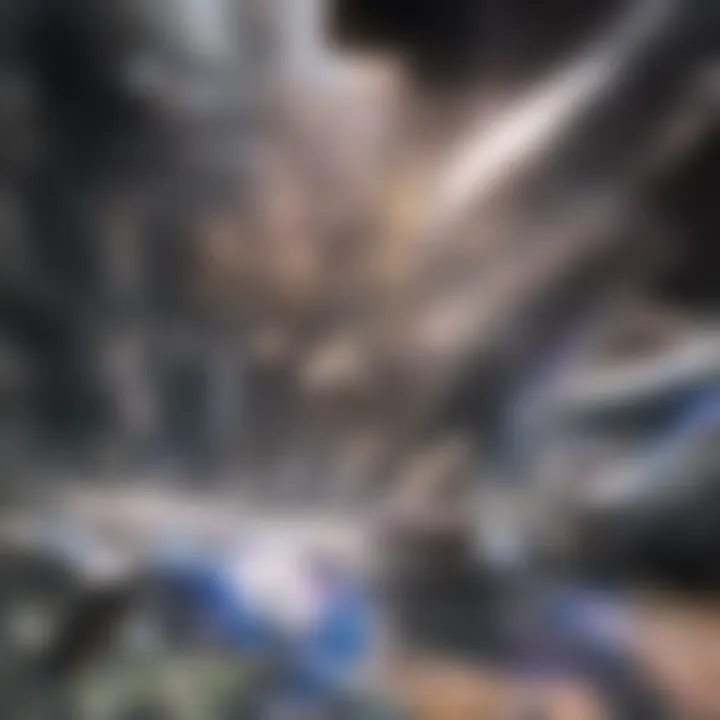
The uncertainty principle, primarily put forth by Werner Heisenberg, states that one cannot simultaneously know both the position and momentum of a particle with absolute precision. The more accurately one property is known, the less accurately the other can be determined. This principle is foundational in distinguishing quantum mechanics from classical physics.
This uncertainty has profound implications for understanding measurement in quantum systems. It suggests that observation itself alters the state of what is being observed, complicating the way we gather data in quantum physics. Researchers in fields such as quantum computing and quantum cryptography must consider these uncertainties in their designs and methodologies. Ultimately, the uncertainty principle challenges the deterministic worldview and introduces a level of unpredictability inherent in quantum systems.
"The quantum world is very strange and unintuitive, revealing the limitations of classical thought in explaining reality."
By unpacking these fundamental concepts, we gain insight into the mechanisms that govern quantum systems. Each principle contributes significantly to our overall understanding of quantum dynamics, guiding technological advancements and offering fresh perspectives on the nature of reality.
Quantum Properties and Classical Physics
Quantum properties fundamentally alter our understanding of physics, especially when compared with classical physics. These differences are pivotal since they challenge long-standing beliefs about matter and energy. Classical mechanics, as derived from Newtonian principles, operates under deterministic laws. In contrast, quantum mechanics introduces a probabilistic nature. This divergence raises important considerations for scientists and researchers, as it necessitates a profound reevaluation of existing theories and models in both theoretical and practical applications.
Differences from Classical Mechanics
The disparities between quantum mechanics and classical mechanics can be characterized through several key aspects:
- Determinism vs. Probabilism: Classical mechanics suggests that if one knows a system's initial conditions, the future can be predicted with certainty. In quantum mechanics, however, predictions are inherently probabilistic. This concept is best illustrated through experiments like the double-slit experiment, where particles exhibit behavior that cannot be accurately predicted using classical physics.
- State and Measurement: In classical mechanics, the state of a system is well-defined. However, in quantum mechanics, the act of measurement plays a critical role. The state of a quantum system can change due to observation, a phenomenon not accounted for in classical paradigms. This leads to what is termed the measurement problem, where measurement can influence reality itself.
- Quantization: In classical physics, energy and other quantities are continuous. In quantum mechanics, these quantities are quantized, meaning they can only take specific values. For instance, electrons in an atom occupy discrete energy levels, contrasting with the continuous energy transitions implied by classical systems.
- Wave-Particle Duality: Classical physics treats particles and waves as distinct entities. Quantum mechanics, however, shows that particles like electrons and photons can demonstrate both behaviors. This duality complicates classical interpretations of electromagnetic radiation and matter.
Understanding these differences is crucial as they inform both theoretical frameworks and experimental approaches in physics. Quantum mechanics introduces complex phenomena that are highly relevant in fields like quantum computing and cryptography.
Complementarity Principle
The complementarity principle, a concept introduced by Niels Bohr, asserts that objects can exhibit particle-like or wave-like behaviors depending on the experimental setup. This idea reflects a fundamental feature of quantum systems: truth may depend on the manner of observation.
- Integrated View: The principle emphasizes that both particle and wave descriptions are necessary to completely understand quantum phenomena. Rather than being competing theories, they complement each other, providing a fuller picture of reality.
- Experimental Implications: This has significant implications in the design of quantum experiments. Observers must choose what aspects to measure, which ultimately influences the outcome.
The complementarity principle furthers our comprehension of quantum behavior, suggesting that these properties cannot be fully grasped without considering potential interactions and measurement conditions.
Applications of Quantum Properties
The applications of quantum properties form a critical intersection between theoretical concepts and practical innovations. Understanding how these concepts translate into usable technologies not only demonstrates the versatility of quantum mechanics but also highlights its potential to revolutionize various domains. This section elaborates on three key areas: quantum computing, quantum cryptography, and quantum sensors. Each of these applications showcases unique benefits and considerations, paving the way for advancements that challenge traditional methodologies.
Quantum Computing
Quantum computing stands at the forefront of technological advancement by harnessing the principles of quantum superposition and entanglement. Unlike classical computers, which utilize bits to process information in binary form, quantum computers utilize quantum bits or qubits. These qubits can exist in multiple states simultaneously, thereby enabling a quantum computer to perform complex calculations at unprecedented speeds.
The potential benefits of quantum computing are substantial. For instance, tasks such as factoring large numbers, simulating quantum systems, and optimizing complex systems can be executed more efficiently than ever before. Notable advancements include Google’s achievement of quantum supremacy in 2019, showcasing the capability of quantum systems to surpass classical performance.
However, significant challenges remain, particularly regarding error rates and decoherence. These issues must be addressed to realize the full potential of quantum computing for practical applications. The continuous research and development in this area signal a promising future for computational power.
Quantum Cryptography
Another prominent application of quantum properties is in quantum cryptography. This field leverages quantum mechanics to enhance security in information exchange. The most widely discussed protocol is Quantum Key Distribution (QKD), which allows two parties to produce a shared, secret random key through the principles of quantum mechanics.
One of the key advantages of quantum cryptography is its ability to provide security against eavesdropping. The act of measuring a quantum state inevitably alters it, revealing the presence of any potential intruder. Consequently, quantum cryptography offers a level of security unattainable through conventional methods.
While the technology shows great potential, considerations regarding implementation and infrastructure must be addressed. The current necessity for direct line-of-sight communication poses limitations, although research into satellite-based QKD, such as the work of the Chinese satellite Micius, is advancing capabilities.
Quantum Sensors
Quantum sensors represent another exciting application of quantum properties. These sensors exploit quantum phenomena to achieve sensitivity levels beyond classical sensors. Applications range from gravitational wave detection to medical imaging and beyond.
For example, atomic clocks, which utilize the vibrations of atoms to measure time with incredible accuracy, demonstrate the precision achievable through quantum mechanics. Moreover, quantum sensors can measure changes in such minute quantities that they can enhance our understanding across various scientific disciplines.
Despite their capabilities, these technologies also face challenges related to environmental factors that can impact performance. Research is ongoing to mitigate these challenges and expand the application of quantum sensors in real-world scenarios.
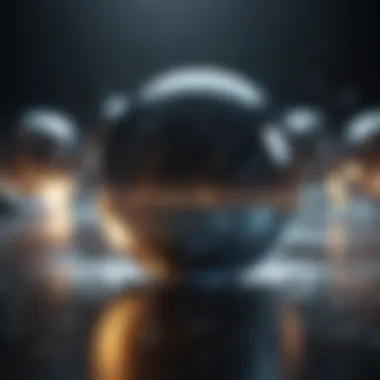
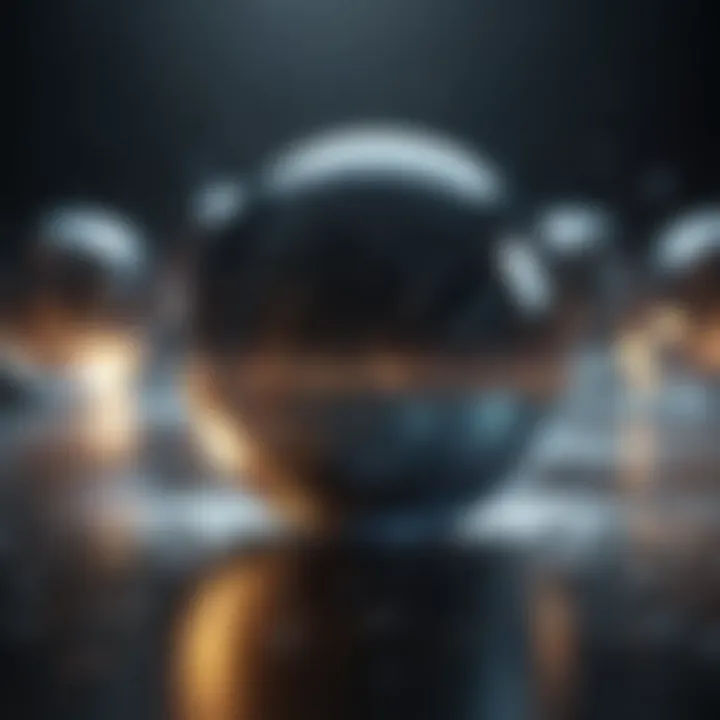
"Quantum technologies are not just theoretical but are progressively being integrated into practical applications that can reshape our future."
In summary, the applications of quantum properties in computing, cryptography, and sensing illustrate a profound shift in capability and understanding. Each application not only represents scientific achievement but also carries significant implications for future research and technology development.
Challenges in Quantum Mechanics
The field of quantum mechanics presents a complex landscape filled with challenges. These challenges not only test the boundaries of current scientific understanding but also spur innovation and technological advancements. Researchers and students in the field must confront various difficulties, from theoretical inconsistencies to experimental limitations, in order to fully grasp the implications of quantum properties. Addressing these challenges is crucial for both the advancement of quantum theory and its practical applications across multiple domains.
Decoherence
Decoherence is a significant challenge in quantum mechanics. It describes how quantum systems interact with their environments, resulting in the loss of quantum coherence. This process is essentially where a quantum system transitions from a state of superposition to a classical state. There lies a fundamental issue: how do we reconcile the quantum world with the classical world we observe daily? Decoherence explains a part of this transition, but it also complicates our understanding of what occurs at a quantum level before this change.
Experiments confirm that decoherence is rapid, often occurring in milliseconds when quantum systems interact with their surroundings. This rapid interaction prevents us from observing phenomena, such as superposition, for extended periods. The importance of controlling decoherence cannot be overstated, especially for applications in quantum computing where maintaining coherence is crucial for operations.
Some key points about decoherence include:
- Loss of Coherence: Quantum states lose their ability to exhibit interference patterns as they interact with their environment.
- Environmental Interaction: Factors like temperature, vibrations, and electromagnetic fields can all contribute to decoherence.
- Implications for Quantum Computing: Understanding and mitigating decoherence is essential for the development of reliable quantum computers.
"Decoherence marks the boundary between the quantum and classical worlds and challenges how we perceive their relationship."
The challenge remains to develop techniques, such as quantum error correction, to counteract the effects of decoherence, which is vital to the advancement of quantum technologies.
Measurement Problem
The measurement problem in quantum mechanics poses another immense challenge. It revolves around the question of how and when a quantum system changes its state due to measurement. When a quantum system is not being observed, it exists in a state of probability. Upon measurement, the system seems to 'choose' a definite state. This phenomenon raises significant philosophical and scientific questions.
A few critical aspects of the measurement problem include:
- Observer Effect: The act of measuring affects the system's response. Thus, the observer plays a crucial role in defining the outcomes of measurements.
- Collapse of the Wave Function: Theories often propose that the wave function collapses to a single eigenstate upon measurement, but the mechanism behind this is not well defined.
- Interpretations of Quantum Mechanics: Various interpretations, such as the Copenhagen interpretation and many-worlds theory, attempt to address the measurement problem, yet no consensus exists.
Understanding the measurement problem is essential not only for fundamental physics but also for the development of practical applications. It influences our conceptual framework of reality and the underlying nature of the quantum world.
In summary, both decoherence and the measurement problem present significant challenges within quantum mechanics. They evoke critical discussions and research in the field, pushing towards a deeper understanding of the universe at its most fundamental level. These challenges shape our approach to quantum technologies and offer fertile ground for future exploration.
Theoretical Implications
The domain of quantum mechanics possesses vast theoretical implications that stretch across multiple scientific disciplines. Understanding these implications is essential to grasp the profound shifts in thought that have emerged from quantum physics. These theoretical frameworks establish the foundations for much of modern physics, influencing the interpretation of events at the subatomic scale and informing us about the nature of reality itself.
One key aspect of theoretical implications is the way they challenge classical intuitions. In classical physics, objects are thought to have definite states and trajectories. However, quantum mechanics fundamentally alters this perception, presenting a reality where particles exist in superpositions and exhibit behaviors that can seem counterintuitive. As such, researchers are compelled to rethink established notions and accept a much more nuanced understanding of physical systems.
Significantly, quantum field theory forms one of the cornerstones of modern theoretical physics. It integrates quantum mechanics with special relativity. This approach treats particles as excitations in underlying fields rather than distinct entities. Consequently, particle interactions become far more complex, requiring advanced mathematical frameworks. The implications of this theory are vast: it leads to the unification of different forces and guides extensive research in particle physics.
"Quantum field theory provides a well-structured language to describe the intricate dance of subatomic particles."
Additionally, the notion of quantum gravity seeks to reconcile general relativity with quantum mechanics. This endeavor is crucial as it attempts to explain gravity in the context of the quantum realm, challenging our understanding of time and space. The implications here are profound, potentially offering insights into the very fabric of the universe.
In summary, the theoretical implications about quantum field theory and quantum gravity push the boundaries of human knowledge. They compel researchers and scientists to re-evaluate traditional paradigms and embrace the unconventional principles that govern the quantum world.
Interdisciplinary Connections
The study of quantum properties extends beyond traditional physics, influencing a variety of fields. Understanding interdisciplinary connections can illuminate the broad impact of quantum mechanics, enriching scientific analysis and technological advancement. This section examines how the principles of quantum mechanics apply to chemistry, biology, and philosophical discussions.
Quantum Properties in Chemistry
In chemistry, quantum mechanics plays a pivotal role in understanding atomic and molecular structures. Quantum mechanics allows chemists to explain how electrons are distributed in atoms and how they interact to form bonds. This understanding is critical when predicting chemical reactions and material behaviors at the molecular level.
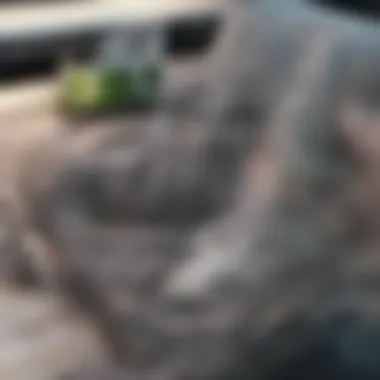
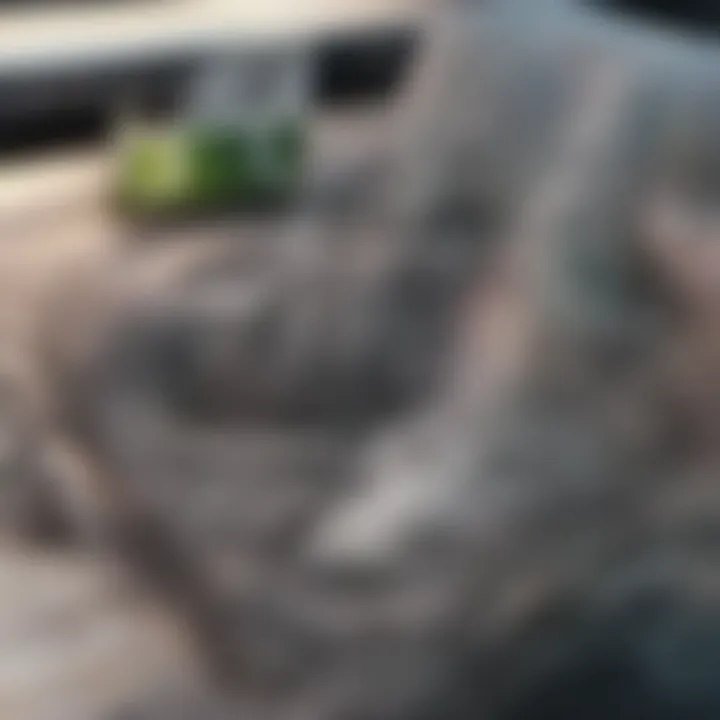
Key concepts include:
- Orbital Theory: This describes the probability distribution of electrons in an atom, essential for predicting how different elements combine.
- Spectroscopy: It relies on quantum properties to analyze how substances absorb or emit light, providing insights into their structure and interactions.
- Quantum Chemistry: A field dedicated to applying quantum mechanics to chemical problems, aiding in the design of new materials and drugs.
Without quantum mechanics, much of modern chemistry would lack the theoretical grounding needed to advance the field.
Quantum Biology
Quantum biology is an emerging field that studies the application of quantum mechanics to biological processes. It investigates how quantum effects influence phenomena such as photosynthesis, avian navigation, and even enzyme activity. This intersection of quantum physics and biology offers potential explanations for complex mechanisms that classical models struggle to address.
Important areas of focus include:
- Photosynthesis: Research suggests that plants may utilize quantum coherence to optimize energy transfer from sunlight.
- Magnetoreception: Certain species are believed to use quantum entanglement to sense Earth's magnetic field for navigation.
- Enzyme Catalysis: Quantum tunneling may play a key role in how enzymes accelerate biochemical reactions.
Understanding these processes can lead to innovations in energy efficiency and biotechnology.
Philosophical Perspectives
The implications of quantum properties inspire rich philosophical discourse, challenging established notions of reality. The concept of superposition raises questions about the nature of existence and observation. Philosophers are increasingly interested in how quantum mechanics influences ideas about determinism, free will, and the role of the observer in the measurement process.
Considerations include:
- The Observer Effect: This phenomenon suggests that the act of observation influences outcomes, prompting debates about the nature of reality.
- Determinism vs. Indeterminism: Quantum mechanics appears to challenge classical determinism, suggesting inherent randomness in quantum events.
- Nature of Consciousness: Some theorists argue that quantum processes could contribute to the understanding of consciousness.
Exploring these philosophical questions adds depth to our understanding of quantum mechanics, revealing its profound implications beyond empirical science.
Understanding the connections between quantum properties and other disciplines can pave the way for groundbreaking discoveries and innovative applications in science and technology.
Future of Quantum Research
The future of quantum research holds significant promise for advancements in various fields. As scientists continue to explore the intricate properties and behaviors of quantum systems, they uncover new potentials that can revolutionize technology, communication, and even our understanding of the universe. The importance of this research is underscored by several specific elements that deserve attention.
Emerging Technologies
Emerging technologies in quantum research are likely to redefine the landscape of various industries. Notable areas include:
- Quantum Computing: This technology harnesses the principles of quantum superposition and entanglement to process information at unprecedented speeds. Companies like IBM and Google are investing heavily in quantum processors that can potentially solve complex problems faster than classical computers.
- Quantum Cryptography: This field focuses on developing secure communication channels using quantum principles. Quantum Key Distribution (QKD) enables two parties to share encryption keys with a guarantee of privacy, thanks to the nature of quantum measurements.
- Quantum Sensing: Utilizing quantum properties enhances measurement precision. Devices such as atomic clocks and gravitational wave detectors are continually improving due to quantum sensing methodologies.
These technologies not only promise increased efficiency and capability but also pose new challenges that require innovative solutions. Researchers strive to bridge theoretical concepts with practical applications, creating a robust foundation for future developments.
Ethical Considerations
As with any transformative technology, ethical considerations in quantum research are essential to address. Some critical points include:
- Privacy and Security: With advancements in quantum cryptography, concerns about data privacy and security emerge. How do we ensure that quantum systems are safeguarded against misuse? Ensuring ethical standards in developing secure technologies is crucial.
- Access and Inequality: As quantum technologies become more prevalent, there is a risk that access may be limited to well-funded organizations and countries. This disparity can lead to a technological divide that exacerbates existing inequalities.
- Scientific Responsibility: The speed at which quantum research is advancing demands that scientists maintain a high level of responsibility. Considering the implications of quantum discoveries on society and the environment is vital for sustainable development.
"The exploration of quantum properties is not merely an academic exercise; it has profound implications for society at large."
End
The conclusion of this article encapsulates the significance of quantum properties in both theoretical and practical realms. Understanding these principles goes beyond mere academic interest; it influences technological advancements and scientific methodologies. In quantum physics, concepts such as superposition and entanglement challenge our classical comprehension of reality. They showcase a world where particles can exist in multiple states simultaneously and where their properties can be interlinked regardless of physical distance.
Summary of Quantum Properties
Quantum properties, including wave-particle duality, quantum superposition, and entanglement, offer a framework for comprehending the intricacies of the universe at a microscopic level. Wave-particle duality reveals that particles like photons exhibit both wave-like and particle-like behavior, a concept that redefines the relationship between light and matter. Quantum superposition allows particles to be in multiple states at once, leading to phenomena that classical physics cannot explain. Entanglement further complicates this picture by demonstrating that particles can become correlated regardless of the distance separating them. Each of these properties not only highlights the limitations of classical theory but also underpins many modern technologies such as quantum computing and cryptography.
Implications for Future Research
The exploration of quantum properties is far from complete. Ongoing research aims to unlock new technologies and deepen our understanding of fundamental physics. Areas like quantum computing promise faster processing and solving complex problems beyond current capabilities. Additionally, quantum cryptography may provide unbreakable communication methods, revolutionizing information security. Future studies should also consider the ethical implications of quantum technologies and how they might reshape our societal landscape. Establishing ethical guidelines now can help mitigate risks associated with new quantum applications. As quantum mechanics continues to progress, its implications will likely expand, bridging gaps between disciplines like biology and chemistry.
The insights gained from quantum research are vital not only for advancing technology but also for comprehending the universe's foundational principles.