Understanding Genetic Inheritance from Parents
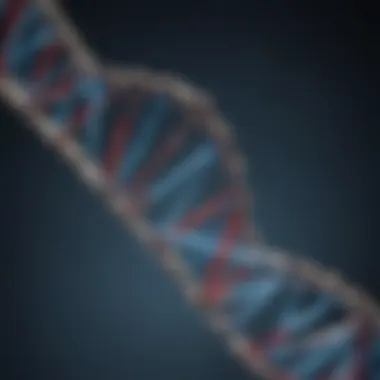
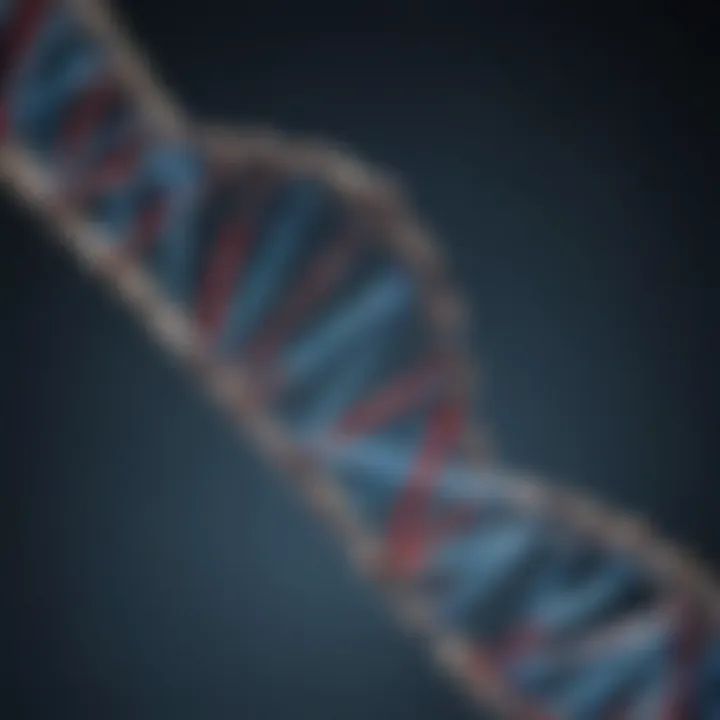
Intro
Genetic inheritance is a fundamental concept in biology that explains how traits are passed from one generation to the next. This process is not only essential for understanding the characteristics of living organisms but also for various applications in health, agriculture, and conservation. The intricate mechanisms that govern this inheritance involve multiple factors, including the DNA structure, alleles, and environmental influences.
Research Overview
The exploration of genetic inheritance reveals several key findings that shed light on its complexities.
Summary of Key Findings
- Role of DNA: DNA carries the genetic information that determines traits. Each gene in the DNA sequence is a blueprint for specific characteristics.
- Dominant and Recessive Traits: Not all genes contribute equally to the phenotype. Dominant traits can overshadow recessive traits, dictating which characteristics are expressed in offspring.
- Environment and Gene Expression: Environmental factors can influence how genes are expressed, leading to variations in traits despite identical genetic makeup.
- Implications for Health: Understanding genetic inheritance helps in identifying genetic disorders and potential hereditary health risks.
Significance of Research
The significance of studying genetic inheritance extends beyond theoretical knowledge. Insights gained from this research play a critical role in fields like medicine, where genetic testing can inform treatment strategies. Additionally, knowledge of inheritance patterns can assist in breeding programs aimed at enhancing desirable traits in crops or livestock.
Methodology
The methodology for studying genetic inheritance encompasses various approaches that allow scientists to observe patterns and behaviors in different populations.
Research Design
Genetic studies typically involve both observational and experimental research designs. Observational designs help identify patterns of inheritance in existing populations. Experimental designs, on the other hand, may include controlled breeding experiments to track the inheritance of specific traits.
Data Collection Methods
Data collection in genetic research often utilizes:
- Phenotypic assessments: Observing physical traits exhibited by organisms.
- Genotypic testing: Analyzing DNA sequences to identify genetic variations.
- Population studies: Examining larger groups to understand how traits are passed down through generations.
Understanding the intricacies of how genes are passed on is fundamental for advancing our knowledge in biology and related disciplines.
Through these methodologies, researchers gain insights into the fundamental principles of genetic inheritance, enhancing the understanding of life sciences.
The Basics of Genetic Inheritance
Understanding the basics of genetic inheritance is essential for grasping how traits are transferred from parents to offspring. It outlines the fundamental mechanics that govern heredity, emphasizing the role of genes, DNA, and chromosomes. This knowledge forms the bedrock of various scientific fields, including genetics, medicine, and biology. Without this understanding, researchers and educators would lack key insights into genetic variations and the implications for health and development.
Genetic inheritance encompasses specific elements like the definition of genes, the structure of DNA, and the organization of chromosomes, each of which plays a critical role in determining an individual's genetic makeup. By exploring these components, one can appreciate how complex traits emerge and how diseases might manifest based on hereditary patterns. This section serves as a primer, prompting deeper exploration into the elaborate mechanisms of genetic transmission.
What Are Genes?
Genes are distinct units of heredity that hold the instructions for creating the proteins vital to the structure, function, and regulation of the body's cells, tissues, and organs. They are segments of DNA located on chromosomes and come in various forms known as alleles. Each gene influences specific traits, contributing to an organism's phenotype, which includes observable characteristics such as eye color and height.
Genes work by coding for proteins that perform specific functions within the body. These proteins can be enzymes, hormones, or structural components. Understanding the role of genes is fundamental in fields like genetic engineering, where modifications can lead to significant advancements in agriculture and medicine.
The Structure of DNA
DNA, or deoxyribonucleic acid, is the molecular blueprint of life. Its double helix structure, characterized by two long strands that wrap around each other, allows for the compact storage of genetic information. Each strand is made up of nucleotides, which are basic units consisting of a phosphate group, a sugar, and a nitrogenous base.
There are four types of nitrogenous bases in DNA: adenine, thymine, cytosine, and guanine. The specific sequences of these bases encode the information necessary for building proteins. The complementary pairing of bases—adenine with thymine and cytosine with guanine—ensures accurate replication during cell division. This fundamental aspect of DNA structure underpins the entire process of genetic inheritance, as it determines how genetic information is passed from one generation to the next.
Chromosomes and Their Role
Chromosomes are tightly packed structures that house DNA within a cell's nucleus. Humans typically have 23 pairs of chromosomes, with one set inherited from each parent. This arrangement allows for the combination of genetic material, which occurs during the fertilization process.
Each chromosome contains many genes, organized linearly. The position of each gene on a chromosome is important because it can influence gene expression and inheritance patterns. For example, an error in the number or structure of chromosomes can lead to genetic disorders, highlighting the importance of chromosomal integrity in inheritance.
In summary, the basics of genetic inheritance provide essential insights into how genes are structured and how they function to affect traits and characteristics in offspring. This knowledge not only enhances our understanding of biology but also informs real-world applications in medicine and genetics.
The Mechanics of Heredity
The mechanics of heredity encompass the processes and systems through which parents transmit genetic material to their offspring. This foundation helps explain the characteristics and traits seen in generations. Understanding these mechanisms is crucial in genetics, as it allows researchers and students to dissect how genetic information is managed and conveyed. The implications are significant for various fields, including medicine, agriculture, and evolutionary biology.
How Parents Pass on Genes
Every parent contributes genes to their children. Each individual has two alleles for every gene, one inherited from each parent. These alleles can be dominant or recessive, influencing physical traits like eye color or susceptibility to certain diseases. The combination of parental alleles determines the genetic blueprint of the offspring. This transmission process ensures genetic continuity, as the genetic makeup is preserved through generations.
The process by which this occurs varies. For many traits, the Mendelian inheritance pattern is observed. However, polygenic inheritance and environmental factors also play essential roles. Understanding this transmission mechanics aids in predicting genetic outcomes.
The Process of Meiosis
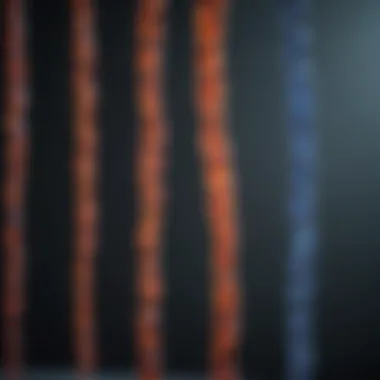
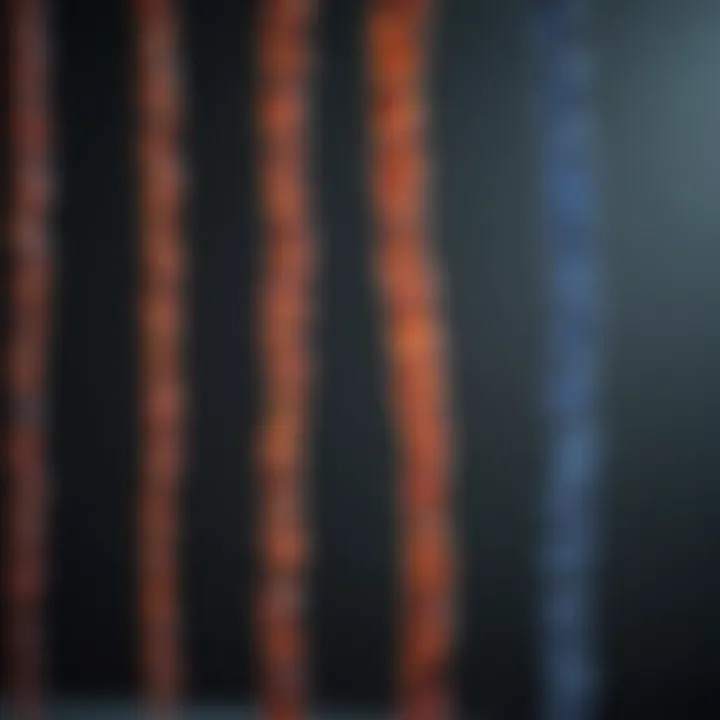
Definition
Meiosis is the specialized form of cell division that occurs in the formation of gametes—sperm and eggs. Unlike mitosis, which creates identical cells, meiosis results in four genetically diverse cells. This diversity is central to sexual reproduction, as it introduces variability to the genetic pool. The unique aspect of meiosis lies in its two rounds of division and the recombination of genetic material, ensuring that offspring exhibit a unique combination of traits inherited from both parents.
Stages of Meiosis
Meiosis consists of several stages, divided into meiosis I and meiosis II. In meiosis I, homologous chromosomes are separated, while in meiosis II, sister chromatids are pulled apart. This structured process allows for precise genetic distribution and any errors can lead to genetic disorders or infertility.
The key characteristic of these stages is the reduction of chromosome numbers by half, ensuring that when gametes fuse during fertilization, the resulting offspring have a complete set of chromosomes.
Recombination
Recombination occurs during prophase I of meiosis. Here, homologous chromosomes exchange segments of genetic material. This exchange is vital for increasing genetic diversity among offspring. The unique feature of recombination is its ability to mix alleles from both parents, leading to new combinations that can enhance adaptability in changing environments.
Recombination is commonly viewed as a beneficial mechanism in the context of evolution. However, it can also result in undesired genetic configurations, leading to inherited genetic disorders.
Fertilization and Genetic Combination
Fertilization is the culmination of meiosis, where the sperm and egg unite. This union creates a zygote with a complete set of chromosomes, half from the mother and half from the father. This process is crucial for the combination of genetic traits, determining the phenotypical and genotypical character of the new individual. It sets the stage for the expression of traits that are influenced by both genetic inheritance and environmental factors.
In summary, the mechanics of heredity provide a pivotal framework for understanding genetic inheritance. It encapsulates how parents pass on genes through meiosis and fertilization, significantly enriching the discourse in genetics and evolutionary biology.
Dominant and Recessive Traits
The topic of dominant and recessive traits is fundamental to the study of genetics and serves as the backbone of how we understand inheritance patterns. Understanding this concept allows researchers, educators, and students to grasp how specific traits are passed on from parents to offspring. This section will elucidate the roles of dominance and recessiveness in genetic inheritance, highlighting why these concepts matter in genetic research and their implications in real-world scenarios.
Understanding Dominance
Dominance refers to the relationship between alleles, which are different forms of a gene. An allele can be dominant or recessive. A dominant allele is one that can mask the effect of a recessive allele when both are present in an individual. For example, if a person has one allele for brown eyes, which is dominant, and one allele for blue eyes, the brown eye trait will be expressed in that individual.
This relationship is important because it helps explain why certain traits are more common in a population.
- Examples of dominant traits include brown eyes, curly hair, and the ability to roll one’s tongue.
- Recognizing dominant traits is essential in fields like genetics and medicine, as it affects the likelihood of developing certain conditions linked to these traits.
The Concept of Recessiveness
Recessiveness is the counterpart to dominance. A recessive allele will only be expressed in an individual's phenotype if both alleles for a specific gene are recessive. If a dominant allele is present, it will override the expression of the recessive allele. This means that many recessive traits may remain hidden or skip generations.
- For instance, blue eyes and straight hair are considered recessive traits.
- In a scenario where both parents carry a recessive allele for blue eyes, there is a possibility that their child can express this trait if the child inherits the recessive allele from both parents.
The understanding of recessiveness is critical in predicting genetic disorders. Certain disorders linked to recessive alleles might not appear in an individual's phenotype but can still be carried and passed on.
Phenotypes and Genotypes
The terms phenotype and genotype are central to genetics and the discussion of dominant and recessive traits.
- Phenotype refers to the observable characteristics of an organism. These are the traits that are visible, such as hair color or height.
- Genotype is the genetic makeup of an organism, indicating which alleles are present, for instance, whether they have one or two dominant or recessive alleles.
By understanding the relationship between genotype and phenotype, one can better predict how traits are inherited. For example, two parents with brown eyes (genotypes Bb and Bb) can have offspring that either have brown eyes or blue eyes based on the combined alleles received from each parent.
Genetic inheritance patterns can be complex, but understanding dominance and recessiveness clarifies how traits are expressed in individuals.
Understanding these concepts not only enriches our comprehension of biology but also emphasizes the complexity of genetic inheritance and its impact on health, behavior, and evolution.
Genetic Variability and Mutations
Genetic variability and mutations play a crucial role in the process of inheritance. They contribute to the diversity of traits seen in populations. This diversity is essential for the survival and adaptation of species. Variability occurs naturally through various mechanisms, including mutations, which can alter the genetic code. These changes in the genome can have significant implications for an organism's phenotype, influencing everything from appearance to disease susceptibility.
Sources of Genetic Variation
Genetic variation stems from multiple sources. For instance, sexual reproduction mixes the genetic material of both parents. This produces offspring with combinations of traits different from either parent. Additionally, mutations are a source of new alleles. They can arise from errors in DNA replication or due to environmental factors. Over time, these variations accumulate and drive the evolutionary process.
Some of the main sources of genetic variation include:
- Crossing Over: This occurs during meiosis, where homologous chromosomes exchange segments of DNA, creating unique genetic combinations.
- Independent Assortment: The random distribution of maternal and paternal chromosomes to gametes generates different allele combinations.
- Random Mutation: Changes in the DNA sequence can result from external factors like radiation or chemical exposure.
The Role of Mutations
Mutations are alterations in DNA that can lead to significant changes in an organism's traits. They serve as the fundamental basis for genetic diversity. Without mutations, evolution and adaptation would not be possible.
Types of Mutations
Mutations can be classified into several types:
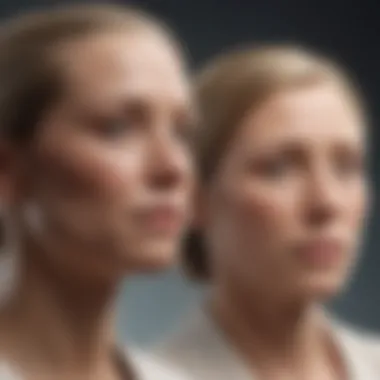
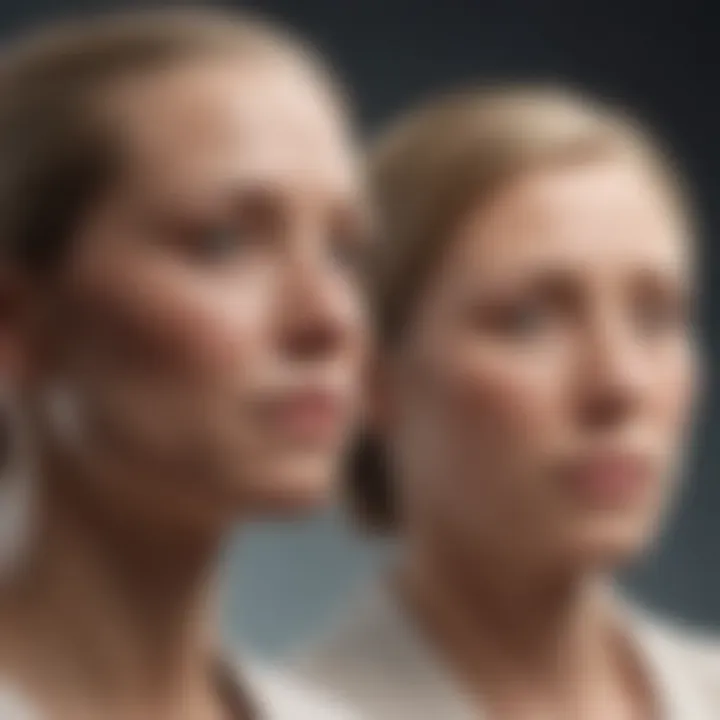
- Point Mutations: These involve a single nucleotide change in the DNA sequence, which can affect protein function.
- Insertions and Deletions: These mutations add or remove nucleotides from the DNA sequence, potentially leading to frameshifts in the genetic code.
- Duplications: This type involves a portion of the DNA being copied, leading to multiple copies of the same gene.
Each type of mutation has unique impacts on the organism. For example, point mutations can have subtle effects, while duplications can create entirely new gene functions. This attribute makes different types of mutations valuable for studying genetic processes. They reveal the complexity and the adaptability of life.
Effects on Genes
The effects of mutations on genes can vary widely. While some mutations are benign, others contribute to disease. Understanding these effects is important for both genetics and medicine.
- Beneficial Mutations: These can enhance an organism's survival or reproduction. For instance, a mutation that provides resistance to a disease can give an organism a significant advantage in survival.
- Neutral Mutations: Many mutations do not affect the organism's fitness. They may remain in the population without noticeable effects.
- Harmful Mutations: These can disrupt normal gene function, leading to genetic disorders or increased susceptibility to diseases.
The study of genetic variability and mutations provides insights into evolution, health, and disease. Understanding their principles is essential for both scientific research and practical applications in medicine.
By studying the sources and effects of genetic variation and mutations, researchers can advance their knowledge on how traits are inherited and expressed. This knowledge is vital for various fields, including genetics, medicine, and evolutionary biology.
The Influence of Environment on Genes
The interplay between genetic inheritance and environmental influences plays a significant role in shaping various traits. Understanding this connection is crucial, as it provides insight into why siblings, despite sharing similar genetic backgrounds, can exhibit different characteristics. The environment affects not only physical traits but also behavioral aspects, leading to diverse outcomes even among closely related individuals. This section explores two key components: epigenetics and environmental factors affecting genes.
Epigenetics Explained
Epigenetics refers to changes in gene expression that occur without alterations to the underlying DNA sequence. These changes can be triggered by various environmental influences, such as nutrition, stress, and exposure to toxins. Through mechanisms like DNA methylation and histone modification, epigenetic factors can turn genes on or off. This means that the genes inherited from parents can behave differently based on an individual’s life experiences.
For instance, a child raised in a nurturing environment may express genes associated with growth and development in healthier ways than a child in a deprived setting. This aspect of epigenetics highlights the dynamic nature of genes. It underscores that hereditary information is not static and can adapt based on external conditions. Moreover, epigenetic changes can sometimes be passed down to future generations, further complicating the inheritance of traits.
Environmental Factors Affecting Genes
Various environmental factors can significantly impact gene expression. These factors include:
- Nutrition: Diet plays a key role in gene expression. Nutrients can affect epigenetic mechanisms, potentially leading to long-term changes in health and development.
- Physical Activity: Regular exercise has been shown to influence gene expression related to metabolic processes and overall health.
- Stress: Chronic stress can lead to changes in gene expression associated with mental health conditions, demonstrating how psychological factors can have biological impacts.
- Exposure to Chemicals: Environmental toxins, such as pesticides or heavy metals, can result in harmful epigenetic changes, influencing disease susceptibility.
- Social Environment: The interactions and relationships that an individual engages in can influence their psychological and emotional health, which in turn can have epigenetic effects.
"The environment provides a context in which genetic potential can either flourish or be suppressed."
By understanding the influence of the environment on genes, researchers and educators can better appreciate the complexities of genetic inheritance. It emphasizes the need for a holistic view when studying traits, considering both genetic and environmental components. This knowledge is also significant for health interventions, suggesting that improving environmental factors can enhance genetic potential and overall well-being.
Genetic Inheritance Patterns
Genetic inheritance patterns are crucial for understanding how traits and characteristics are transferred from parents to offspring. The study of these patterns provides insight into both simple and complex traits, allowing researchers to predict genetic outcomes based on parental lineage. Mendelian inheritance forms the foundation of these patterns, elucidating principles such as segregation and independent assortment, which are essential for grasping basic genetic concepts.
Moreover, recognizing non-Mendelian inheritance patterns broadens the scope of genetic analysis. These patterns include phenomena such as incomplete dominance, codominance, and polygenic inheritance. Each of these characteristics contributes significantly to the genetic diversity observed in populations, a vital aspect of evolutionary biology.
Understanding genetic inheritance patterns encourages informed decisions in various fields, including genetics, medicine, and agriculture. This knowledge can guide genetic testing, breeding strategies, and the assessment of hereditary diseases. The benefits of mastering these concepts extend beyond academic interest; they are applicable in real-world scenarios affecting health, agriculture, and biodiversity.
Mendelian Inheritance
Mendelian inheritance is based on the work of Gregor Mendel, who discovered fundamental principles of inheritance through his work with pea plants. His observations led to the formulation of two key laws. The first is the Law of Segregation, which states that alleles for a trait separate during gamete formation, ensuring offspring inherit one allele from each parent. The second is the Law of Independent Assortment, which indicates that alleles for different traits are distributed to gametes independently.
Mendelian inheritance is significant because it provides a structured approach to genetic predictions. By understanding dominant and recessive traits, scientists can predict the likelihood of certain traits appearing in offspring. This clarity is particularly beneficial in fields such as horticulture and animal breeding, where specific traits are desired.
Non-Mendelian Inheritance
Non-Mendelian inheritance patterns expand on Mendelian principles to encompass more complex genetic behaviors.
Incomplete Dominance
Incomplete dominance is a form of inheritance where neither allele is completely dominant over the other. This results in a phenotype that is a blend of both parents' traits. For example, when a red flower is crossed with a white flower, the offspring may produce pink flowers.
This blending of traits is essential because it illustrates that the inheritance of genes is not always straightforward. It adds a layer of complexity to our understanding of genetic diversity and expression. Incomplete dominance is a vital component of this article because it encourages deeper investigation into how traits are visually represented and can create new phenotypes.
Codominance
Codominance is another intriguing inheritance pattern, where both alleles contribute equally to the phenotype. A classic example is seen in certain breeds of cattle, where the offspring can exhibit both red and white patches when a red cow is crossed with a white cow.
This aspect shows that genetics can produce a variety of outcomes, emphasizing the beauty of diverse expressions in traits. Codominance is essential for understanding how mixed populations of genes interact, adding nuance to the study of trait inheritance.
Polygenic Inheritance
Polygenic inheritance involves multiple genes determining a single trait, such as skin color or height in humans. Each contributing gene has a small but additive effect on the phenotype, leading to a wide range of possible outcomes.
This characteristic of polygenic inheritance highlights the complexity involved in predicting traits, as many factors influence the final outcome. The broad spectrum of phenotypes in traits governed by multiple genes makes this concept crucial for the overall discussion in this article. In practical terms, polygenic inheritance affects everything from agricultural yields to human health outcomes, illustrating its real-world relevance.
Genetic inheritance patterns are more than just a means of understanding biology; they are the key to unlocking mysteries of heredity, disease, and diversity in all forms of life.
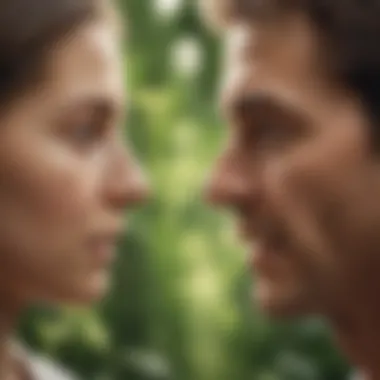
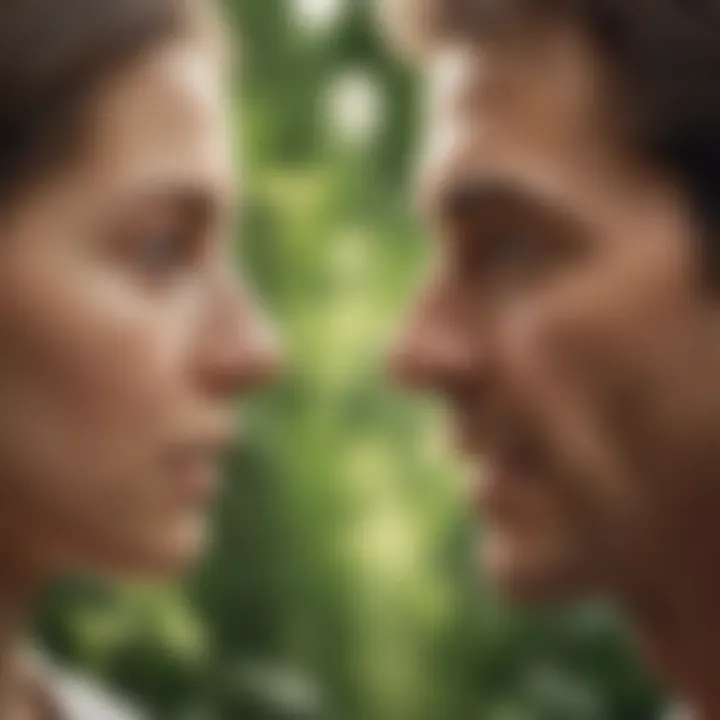
Applications of Genetic Knowledge
Understanding genetic inheritance has vast applications that extend across multiple disciplines. In medicine, farm production, and understanding human behavior, the implications are significant. Genetic knowledge allows for targeted therapies, improved crop strains, and insights into hereditary diseases, while also prompting ethical discussions. This section will elaborate on several key areas where this knowledge finds practical application.
Implications for Health and Medicine
Advancements in genetic knowledge play a crucial role in preventive health care and treatment of diseases. With a better understanding of how genes influence our health, medical professionals can identify genetic predispositions to certain conditions. This enables them to create personalized treatment plans. Moreover, awareness of family history in genetic diseases can inform screening procedures. For example, individuals with a family background of breast cancer might benefit from genetic counseling or BRCA testing to consider preventive measures.
Genetic Testing and Screening
Genetic testing and screening help in the early identification of disorders. The methodologies used can vary significantly in purpose.
Types of Tests
There are several types of genetic tests, each serving a distinct purpose. These include:
- Diagnostic Testing: This is performed to confirm or rule out a specific genetic or chromosomal condition.
- Carrier Testing: Identifies if a person carries a gene for a recessive disorder. This is especially vital for prospective parents.
- Prenatal Testing: Used to detect changes in a fetus's genes or chromosomes before birth.
- Newborn Screening: Conducted shortly after birth to identify genetic disorders that require immediate attention.
Each type has significant benefits. Carrier testing, for instance, helps prospective parents make informed decisions about family planning. Prenatal testing can prompt early interventions, which can be life-saving.
Benefits and Limitations
While genetic testing provides many benefits, it also has limitations.
- Benefits: Early detection of diseases, customized treatments, and knowledge for future family planning are among the major advantages. Enhanced screening can lead to better health outcomes and quality of life.
- Limitations: False positives or negatives can occur, leading to unnecessary anxiety or false security. Furthermore, ethical dilemmas arise concerning genetic privacy and potential discrimination based on test results.
Gene Therapy and Genetic Engineering
Gene therapy is an innovative application of genetic knowledge that involves altering genes within an individual’s cells to treat or prevent disease. It holds immense promise for various genetic disorders, including some cancers, cystic fibrosis, and hemophilia. As technology advances, so do the techniques in gene editing, like CRISPR-Cas9, which allow for precise modifications to the DNA sequence. This opens discussions about not only the therapeutic potential but also the ethical ramifications of altering the human genome. The balance between beneficial medical interventions and moral considerations continues to be a challenging but essential conversation.
Ethical Considerations in Genetics
Ethical considerations in genetics have become increasingly prominent in research and societal discussions, particularly as advancements in technology continue to rapidly evolve. Understanding these ethical aspects is essential for responsibly navigating the complexities associated with genetic manipulation and data privacy.
Genetic manipulation refers to altering the genetic makeup of organisms, which raises questions regarding its applications, potential consequences, and moral implications. These considerations hinge on values such as fairness, justice, and respect for life.
One significant benefit of addressing ethical issues is the establishment of frameworks that guide genetic research, ensuring it aligns with societal values. Additionally, a robust ethical understanding can improve public trust in biotechnologies, essential for their acceptance and integration into daily life.
The Ethics of Genetic Manipulation
Gene editing technologies, such as CRISPR-Cas9, offer powerful tools for modifying organisms' genomes. This potential is not without controversy. The ethics surrounding genetic manipulation often focus on various fundamental questions, including:
- Should we manipulate human genes?
- What are the long-term ecological impacts of genetically modified organisms?
- How do we ensure equitable access to these technologies?
Many advocate for careful regulation, promoting responsible research while considering possible unintended consequences. Critics argue that playing with the fundamental blueprint of life can lead to ethical dilemmas, especially concerning designer babies and potentially exacerbating social inequalities.
Privacy Concerns in Genetic Data
As more individuals undergo genetic testing, the privacy of genetic information has become a pressing concern. Genetic data holds sensitive information about predispositions to disease and other personal characteristics.
One of the main challenges is ensuring confidentiality while providing useful insights to individuals. Concerns include:
- Data security risks: Unauthorized access to genetic data can lead to misuse, including discrimination by employers or insurance companies.
- Informed consent: Participants often may not fully understand what they agree to when sharing their genetic material, which can lead to ethical backwardness.
To address these issues, robust data protection policies and ethical standards are crucial. The implementation of guidelines that govern data sharing, storage, and individual rights can enhance trust and promote ethical practices within genetic research.
Protecting individual privacy in genetic data is as crucial as advancing our scientific understanding, ensuring that research benefits society without compromising personal rights.
Future of Genetic Research
As we delve into the future of genetic research, it becomes increasingly clear that this is a dynamic frontier in the realm of biology and medicine. The ongoing advances in genetic technologies promise not only to enhance our understanding of genetic inheritance but also to transform various sectors, including healthcare, agriculture, and biotechnology. This section explores specific elements, benefits, and considerations associated with the future of genetic research, emphasizing its significant relevance in our lives and society.
Advancements in Genetic Technologies
Recent years have witnessed remarkable strides in genetic technologies. Techniques such as CRISPR-Cas9 gene editing, next-generation sequencing, and RNA interference have revolutionized the way scientists approach genetic research.
- CRISPR-Cas9 allows for precise editing of DNA, enabling researchers to modify genes in various organisms. This technology has vast potential for correcting genetic disorders and improving agricultural practices.
- Next-generation sequencing facilitates the rapid sequencing of genomes at a lower cost, allowing for extensive studies across populations. This advancement assists in identifying genetic predispositions to diseases.
- RNA interference is a method for silencing specific genes, which helps in examining gene function and its implications on health.
These advancements not only deepen our understanding of genetic mechanisms but also open doors to personalized medicine, where treatments can be tailored to an individual's genetic profile.
Potential Impact on Society
The implications of advanced genetic research extend far beyond the laboratory.
- Healthcare: By identifying genetic risk factors, healthcare providers could personalize treatments, reducing ineffective interventions while maximizing positive outcomes. This shift could significantly improve patient quality of life and healthcare efficiency.
- Food Production: Genetic advancements can lead to crop varieties that are resistant to pests and diseases, enhancing food security. The use of genetically modified organisms (GMOs) continues to spark debate but also holds potential for sustainable agriculture in the face of climate change.
- Ethical Considerations: As with any powerful tool, the advancements in genetic technologies raise ethical questions. The ability to modify genes invites discussions about designer babies, genetic privacy, and the consequences of playing with the building blocks of life.
"Understanding the ethical dimensions of genetic research is essential for navigating its potential and limitations successfully."
In summary, the future of genetic research is laden with promise and urgency. By harnessing the technologies and insights that continue to emerge, society can work to address pressing challenges. The path forward must balance innovation with consideration of ethical implications. Genetic research stands at the intersection of possibility and responsibility, ushering in changes that could significantly reshape our world.